ESCHERICHIA COLI INFECTION IN POULTRY
Nguyen Pham Thao Nhi, Tran Duy Thanh
Nguyen Khanh Thuan, Nguyen Phuc Khanh, Nguyen Thanh Lam*
1. Introduction
Colibacillosis refers to any localized or systemic infection caused entirely or partly by avian pathogenic Escherichia coli. Avian pathogenic Escherichia coli (APEC), an extra-intestinal pathogenic E. coli (ExPEC), causes diverse local and systemic infections in poultry, including chickens, turkeys, ducks, and many other avian species. The most common infections caused by APEC in chickens are perihepatitis, airsacculitis, pericarditis, egg peritonitis, salphingitis, coligranuloma, omphalitis, cellulitis, and osteomyelitis/arthritis; these are commonly referred as avian colibacillosis (Dziva and Stevens 2008). APEC also causes swollen head syndrome in chickens and osteomyelitis complex in turkeys (Dziva and Stevens 2008). Colibacillosis is one of the leading causes of mortality (up to 20%) and morbidity in poultry and also results in decreased meat (2% decline in live weight, 2.7% deterioration in feed conversion ratio) and egg production (up to 20%), decreased hatching rates, and increased condemnation of carcasses (up to 43%) at slaughter (Dho-Moulin and Fairbrother 1999, Mellata 2013, Guabiraba and Schouler 2015). Furthermore, APEC is responsible for high mortality (up to 53.5%) in young chickens (Mellata 2013).
2. Aetiology
2.1 Bacteria characteristics
E. coli is a gram negative, non-acid-fast, non-spore forming bacterium variable in size and shape usually 2-3 x 0.6 µm. It normally inhabits the intestinal tract of all animals and birds. There are a number of different strains and many are species-specific. Most strains are motile with peritrichous flagella.
E. coli can grow both aerobically and anaerobically, and uses simple carbon and nitrogen sources. The bacterium grows on ordinary nutrient media at temperature of 18-44°C. On nutrient agar plates, incubated for 24 hours at 37°C, colonies are low, convex, smooth and colorless. It rapidly produces turbidity in broth culture. E. coli possesses three types of antigens: ‘O’ (somatic), ‘H’ (flagellar) and ‘K’ (capsular). ‘O’ antigen is a heat resistant endotoxin liberated on lysis of bacteria and is a major antigen responsible for classification of E. coli. ‘K’ antigen is associated with virulence. ‘H’ and ‘K’ antigens are heat labile. Sixty per cent of the cases are caused by group ‘O’. Pathogenic and nonpathogenic E. coli can be differentiated by Congo red dye where pathogenic isolates produce red colonies. They grow in presence of low concentration of iron and cause hemolysis on blood agar, show adherence with pili to epithelial cells and demonstrate pathogenicity in 3 weeks chicks. Around 10-15% of the intestinal coliforms belong to pathogenic serotypes. The disease causes decreased growth rate, depressed feed conversion efficiency, elevated flock mortality, downgrading and subsequent low performance in infected birds. E. coli is a member of the normal microflora of the poultry intestine, but certain strains - APEC, spread to various internal organs and cause colibacillosis characterized by systemic fatal disease. APEC forms certain serogroups, particularly O93, O92, O78, O1, and O2 and to some extent O15 and O55 are the predominant serogroups. APEC probably does not cause intestinal diseases. Nevertheless, ETEC are occasionally isolated from poultry suffering from diarrhea, and diarrhea was also experimentally induced after intramuscular inoculation of APEC. Enteropthogenic E. coli (EPEC) were isolated from clinically healthy chickens. Isolates of certain O-types are very much heterogenous (both in terms of phenotypes as well as genotypes) although they are detected more frequently in APEC than in commensal E. coli (Kuldeep, Sandip et al. 2013).
Virulence factors
The idea that avian colibacillosis is a secondary disease and APEC are opportunists is widely accepted. However, increasing evidence indicates that most APEC are well equipped for a pathogenic lifestyle, suggesting that APEC infections might not always be opportunistic or secondary to some predisposing condition. Certainly APEC, like other pathogenic E. coli, have acquired genes by horizontal transfer that encode virulence factors, which serve to distinguish APEC from commensal strains. These virulence genes may be clustered into chromosomal or plasmid located pathogenicity islands (PAIs). Because APEC usually cause extraintestinal disease, they are commonly classified as a subpathotype of the ExPEC pathotype. The ExPEC pathotype also includes uropathogenic E. coli (UPEC) and neonatal meningitis E. coli (NMEC) that cause disease in humans and other hosts. ExPEC share certain virulence attributes enabling their extraintestinal lifestyle, including adhesins, toxins, protectins, iron acquisition mechanisms, and invasins. Identification of these traits among APEC has fostered development of a rudimentary definition of an APEC pathotype and led to interest in APEC’s zoonotic potential.
Despite the fact that most APEC infections are extraintestinal, some APEC contain traits associated with intestinal E. coli pathotypes, including EPEC, ETEC, enteroinvasive E. coli (EIEC), enterohemorrhagic E. coli (EHEC), and enteroaggregative E. coli (EAEC, EAggEC). Furthermore, APEC strains causing the same disease may differ substantially in their gene content. In view of this high level of genomic plasticity, it is not surprising that no single virulence factor has been identified that will distinguish all APEC from all commensal E. coli strains. However, APEC’s plasmid PAIs occur so commonly among APEC that they are considered a defining trait of the APEC pathotype, whose presence in an avian E. coli isolate has been used diagnostically (McMullin 2020). Table 1 provides the list of virulence and pathogenesis factors defined or characterized in APEC to date along with their roles in APEC pathogenesis/infection.
Table 1. APEC virulence and pathogenesis factors and their role in systemic infections (Kathayat, Lokesh et al. 2021).
Virulence factors | Genes/Proteins involved | Role in pathogenesis/infection |
Adhesins | fimH, fimC, papA, papC, papEF, papG I, papG II, papGIII, felA, sfa/sfaS, afaIBC, focGE, lpfA, lpf 0141, lpf 0154, flgE, crl, csg, bmaE, tsh, mat/ecpA, hra/hrlA/hek, iha, yqiG, kii | Adhesion, colonization, biofilm formation, motility, intracellular survival |
yfc O | Adhesion, colonization, resistance to environmental stresses | |
yad C | Adhesion, intracellular survival, motility | |
aat A, aatB, upaB | Adhesion, colonization, biofilm formation | |
fdtA, rluD, yjhB, ecpR, fdeC | Adhesion | |
Invasins | ibeA, ibeB, tia, gimB | Invasion, resistance to oxidative stress, colonization, proliferation, biofilm formation |
IbeR | Invasion, resistance to serum and environmental stresses, expression of virulence genes | |
ych O | Motility, adhesion, invasion, biofilm formation, expression of membrane proteins and metabolism genes | |
Iron acquisition systems | iutA, iucC, iucD, aerJ, iucA, iucB, iroBCDEN, fyuA, sitABCD, mntH, feoB, irp2, ireA, eitABCD, fepC, chuA, bfr | Iron and manganese uptake from the host, adhesion, invasion, colonization, persistence, expression of virulence genes, resistance to environmental stresses |
entE, entS, tolC | Invasion, colonization, persistence | |
Protectins | iss, traT, ompT, kpsMT(K1), kpsMT(II), kpsMT(III), neuC, neuS, neuD, kfiC-K5, betA | Protect from serum bactericidal activity and phagocytosis, adhesion, invasion, intracellular survival, colonization, proliferation |
YbjX, PagP | Resistance to serum and environmental stresses, invasion, intracellular survival | |
OmpA | Intracellular survival | |
wzy | Adhesion, invasion, intracellular survival, colonization | |
waa L | Motility, resistance to phagocytosis and environmental stresses, adhesion, invasion, biofilm formation | |
sod A | Protect against ROS-mediated host defenses, biofilm formation | |
lpx M | Invasion, intracellular survival, colonization, regulation of expression of cytokine genes and nitric oxide production | |
Toxins | hlyF, hlyA, hlyE, cdtB, cdtS, vat, sat, stx2f, astA, pic, EAST-1, espC, ace4/35 | Cell lysis and damage, induce host cell vacuolization, colonization, motility, biofilm formation, agglutination, formation of outer membrane vesicles |
Other virulence and pathogenesis factors | ||
Quorum-sensing system (AI-2) | LuxS, LsrABCDFGK, ptsI, Pfs | Motility, biofilm formation, adherence, invasion, colonization, intracellular survival, persistence, expression of virulence genes, cell damage |
Secretion systems | DotU, CpxRA, IcmF, Hcp, ClpV, VrgG (Type VI) | Interbacterial competition, adhesion, invasion, intracellular survival, colonization, motility, biofilm formation, production of type 1 fimbriae, resistance to serum bactericidal activity, modulation of intracellular host responses (IL-18, IL-1β) |
EtrA, YqeI, EivC (Type III) | Motility, intracellular survival, resistance to phagocytosis and serum bactericidal activity, proliferation, expression of fimbriae genes, downregulation of pro-inflammatory cytokines | |
Two-component systems | PhoPQ, tolC | Biofilm formation, motility, adhesion, invasion, intracellular survival, systemic infection, expression of virulence genes and genes associated with flagellar assembly, ABC transporters, quorum sensing, and bacterial chemotaxis |
BasSR | Biofilm formation, APEC virulence and colonization in vivo | |
KdpDE | Expression of flagella-related genes, flagellum formation, motility and resistance to serum bactericidal activity | |
RstAB, hdeD | Iron acquisition, acid resistance, intracellular survival, colonization | |
BarA-UvrY | Adhesion, invasion, persistence, intracellular survival, resistance to serum bactericidal activity and oxidative stress, regulation of exopolysaccharide production and type 1 and P fimbriae | |
Transcriptional regulators | AutA/AutR | Expression of K1 capsule and acid resistance systems, adaptive lifestyle change |
FNR | Adhesion, invasion, expression of type 1 fimbriae and type VII secretion system, resistance to oxidative stress | |
YjjQ | Flagellar motility | |
McbR | Biofilm formation, response to H2O2 | |
tyrR | Invasion, motility, intracellular survival | |
RfaH | Invasion, intracellular survival, resistance to serum bactericidal activity | |
Metabolism-associated genes | acs -yjcH-actP | Intracellular survival, proliferation, colonization, production of pro-inflammatory cytokines and nitric oxide |
PotE, PotF | Colonization, adhesion | |
NirC | Adhesion, colonization | |
ArcA | Chemotaxis, motility | |
Miscellaneous | OmpF, OmpC | Adhesion, invasion, colonization, proliferation |
Prophage phiv142-3 (orf 20) and phiv205-1 | Resistance to serum and environmental stresses, adhesion, invasion, intracellular survival, colonization, biofilm formation, formation of flagella and I fimbriae | |
YicS | Motility, biofilm formation, invasion | |
cpd B | Colonization | |
pst B | Resistance to serum bactericidal activity and oxidative stress, colonization | |
tmRNA-SmpB | Colonization, persistence, replication, intracellular survival | |
mli C | Resistance to serum bactericidal activity | |
malX, frz, cvaABC, cvi, cba, cib/cibI, cbi, cma, eaeA, sopB, yfcV, gad, mchBCF, mcmA, bor, air, eilA, celB, pabB, capU, cif, tir, tccp, nleB, iaL, cjrC, mig-14p | Unknown/not clearly known functions | |
Genes essential for systemic infections and adaptation | metH, lysA, pntA, purL, serS, ybjE, ycdK (rutC), wcaJ, gspL, sdsR, irp2, eitD, ylbE, yjiY, tkt1, pilN, pilQ, tsh, hpb, TcfD, Z5222, waaO, waaY, iutA, iucA, iucD, iroC, ColE2, traK, traG, traT, SopA, psiA, hkaG, hkbV, hkbQ, Z3370, Int, CC0532, TM0427, YPO3000, rhsH, RSp0733, bioABFCD, rnfA, rfnE, gene encoding endonuclease III, creABCD, yehD, potF, flgE, tyrR, bfr | Systemic APEC infections and adaptation
|
2.2 Classification
Escherichia is the type genus of the family Enterobacteriaceae, which is composed of organisms that can grow aerobically or anaerobically and utilize simple carbon and nitrogen sources. E. coli is the type species of the genus Escherichia. Additional species have been assigned to the genus but E. coli occurs most commonly and is most important as a pathogen. Although Shigella is still recognized as a genus with 4 species, they actually group genetically with E. coli (McMullin 2020).
2.3 Pathogenicity
The ability to cause mortality in embryos or chicks differentiates APEC from commensal E. coli strains (McMullin 2020). An embryo lethality test can be used to test avian E. coli isolates for virulence. Eleven 12‐day‐old chicken embryos are inoculated via the allantoic cavity with 100 cfu of the test organism. Two‐day mortality is less than 10% for nonvirulent strains, 10–29% for intermediate strains, and more than 29% for virulent strains (Wooley, Gibbs et al. 2000). Extending the postinoculation observation time resulted in higher mortality, but the pattern of mortality among various strains remained essentially unchanged (Montgomery, Jones et al. 2005). Intravenous and subcutaneous inoculation of chicks correlated with embryo lethality, whereas intratracheal inoculation did not (Gibbs, Petermann et al. 2004). Compared with the embryo lethality test, virulence of an isolate correlated with complement resistance and the presence of the ColV plasmid, but neither of these tests conclusively identified all isolates as virulent strains. Efforts to differentiate APEC from commensal E. coli using in vitro tests have proven effective in some strains. Efforts to differentiate APEC from commensal E. coli using in vitro tests have proven effective in some strains (McMullin 2020).
3. History
Mortality of fowls and isolation of a bacterium from heart, liver, and spleen that was consistent with E. coli was first reported by Lignieres in 1894 (Palmer and Baker 1923). Following experimental inoculation, the isolate was virulent for pigeons and variably virulent for chickens depending on dose and route of administration. Subsequently diseases in grouse, pigeons, swans, turkeys, quail, and additional chicken flocks associated with a similar organism were documented between 1894 and 1922 (Palmer and Baker 1923). The first description of colisepticemia was published in 1907 based on chickens dying from a cholera‐like disease while being transported (Palmer and Baker 1923).
Infectious enteritis and paralysis from which E. coli could be isolated was described in 1923 (Palmer and Baker 1923). In 1938, a pullorum‐like disease associated with poor incubation was reported in chicks less than 10 days of age diagnosed with pericarditis, perihepatitis, and white spots on the liver with E. coli isolated from tissues. By 1965, E. coli had been isolated from a variety of lesions affecting virtually all bird organs, as well as eggs (Sojka 1965).
4. Epidemiology
4.1 Geographic distribution
4.2 Susceptible hosts
Most, if not all, avian species are susceptible to colibacillosis. The various forms of colibacillosis are considered to be the most common infectious bacterial disease of broiler chickens and turkeys. Natural infections of other avian species occur including quail (Burns, Otalora et al. 2003), pheasant (Swarbrick 1985), pigeons (Raue, Schmidt et al. 2005), guinea fowl (Litjens, van Willigen et al. 1989), waterfowl (Bisgaard 1995), (Crespo, Walker et al. 2001), (Miller, Hatkin et al. 2004), ostriches (Knöbl, Baccaro et al. 2001), emus (Hines, Styer et al. 1995), peacocks (Barbieri, Tejkowski et al. 2012), and partridge (Díaz-Sánchez, Sánchez et al. 2012), especially if they are kept intensively in confined conditions. The disease is less common in wild birds (Hoque, Burgess et al. 2012).
Age of host commonly affected
All ages are susceptible to colibacillosis, but young birds are more frequently affected and disease severity is greater in young birds, including developing embryos (Harry 1957, Montgomery, Boyle et al. 1999, Johnson, Bilgili et al. 2001). Outbreaks can occur in caged layers (Zanella, Alborali et al. 2000, Vandekerchove, Vandemaele et al. 2005) and coliform salpingitis/peritonitis is a common cause of mortality in breeders (Jordan, Williams et al. 2005). Colibacillosis in older birds is often manifested as an acute septicemia.
Host susceptibility factors
Compared with bacterial virulence factors, host susceptibility and resistance factors are probably an equal or greater determinant of colibacillosis occurrence. Normal, healthy birds with intact defenses are remarkably resistant to naturally occurring E. coli exposure including virulent strains. Infection occurs when skin or mucosal barriers are compromised (e.g., unhealed navel, wounds, mucosal damage from viral, bacterial, or parasitic infections, lack of normal microbiota, etc.), the mononuclear‐phagocytic system is impaired (e.g., viral infections, toxins, nutritional deficiencies), there is immunosuppression (e.g., viral infections, toxins), exposure and/or stress are overwhelming (e.g., environmental contamination, wide temperature variation, poor ventilation, contaminated water). Effective control of colibacillosis depends on identifying and eliminating the predisposing cause(s) of the disease (McMullin 2020).
4.3 Transmission
APEC invades the gastrointestinal and respiratory tracts through abraded tracheal and intestinal epithelium in the presence of stressors and reaches bloodstream and internal organs. Chickens get infected through contaminated feed and water and can spread to other birds through the feco-oral or aerosol route. Furthermore, APEC can be vertically transmitted from infected breeders via contaminated eggs (Kathayat, Lokesh et al. 2021).
5. Pathogenesis
E. coli enters host tissues following mucosal colonization or directly through breaks or openings in the skin. Mucosal colonization is dependent on adhesin factors that permit the bacterium to attach to receptors and subsequently reproduce. A variety of fimbrial and nonfimbrial adhesins are produced by E. coli, which facilitates their attachment to host cells. There is good evidence that 2 fimbriae (Type 1 [F] and P fimbriae) are important in the initial stages of infection. Type 1 fimbriae are expressed by E. coli that attach to upper tracheal epithelium (Pourbakhsh, Dho-Moulin et al. 1997), oviductal epithelium, and digestive tract mucosa (Elfadil, Vaillancourt et al. 1996). P fimbriae are expressed in deeper tissues (Pourbakhsh, Dho-Moulin et al. 1997). Type 1 fimbriae bind to mucus in the digestive tract but not to goblet cells producing the mucus. In contrast, AC/I fimbriae bind poorly to mucus but attach to goblet cells (Edelman, Leskelä et al. 2003). Flagella aid in penetrating the mucous layer in order to reach the cell surface, and curli, another adhesin factor, aids in attachment to the cell surface (La Ragione, Sayers et al. 2000).
Virulent strains are capable of traversing the mucosa, especially if an injurious agent has compromised it, and surviving within the internal milieu of the body. Exactly how E. coli crosses mucosal barriers is poorly understood. Bacteria may penetrate between damaged cells.
Air sac epithelial cells round up and become vacuolated following exposure to virulent strains, which causes them to separate from each other providing bacteria access to systemic tissues (DeRosa, Ficken et al. 1992, Pourbakhsh, Boulianne et al. 1997). The ability of APEC to bind with fibronectin and laminin, 2 components of basement membranes, would aid in penetration through the damaged mucosa into host tissues (Ramírez, Almanza et al. 2009). Toxins in cell‐free culture filtrates, most likely endotoxin, produce the same acute inflammatory response as the living organism (DeRosa, Ficken et al. 1992).
Alternatively, the initial portal of entry into the host’s tissues, an essential first step in colisepticemia, may be transcellular through nonphagocytic cells. Certain strains of APEC have the ability to invade fibroblasts, much like virulent S. typhimurium (Matter, Barbieri et al. 2011). E. coli have been identified within air sac epithelial cells by electron microscopy(Pourbakhsh, Boulianne et al. 1997). An APEC strain was able to readily adhere to and invade tracheal epithelial cells in primary cell culture and tracheal explants (Ramírez, Almanza et al. 2009). Factors involved in cell penetration remain to be identified. Once E. coli becomes extramucosal, the environment it has entered is extremely hostile. Unless the organism is equipped with survival capabilities (e.g., “virulence” factors), it is rapidly destroyed by phagocytic cells such as heterophils, thrombocytes, and macrophages (Harmon and Glisson 1989, Harmon 1998, Horn, Corrêa et al. 2012). Macrophages located primarily in the spleen and liver phagocytize bacteria that gain access to the circulation. Complement and antibodies to O antigens (endotoxin), outer‐membrane proteins (siderophores), and fimbriae serve as opsonins to promote phagocytosis and destruction of the organism. Endotoxin also decreases the bacteriocidal ability of pulmonary macrophages, which may aid in survival and dissemination. In vitro results suggest that the dying cells in the lung may be macrophages as well as heterophils (Horn, Corrêa et al. 2012). Immediately after E. coli contacts host tissues, there is an acute inflammatory response. Acute phase proteins produced in the liver and cytokines IL‐1, IL‐6, and tumor necrosis factor increase rapidly following exposure to endotoxin or E. coli, which can serve as nonspecific indicators of early disease (Nakamura, Mitarai et al. 1998, Chamanza, van Veenm et al. 1999, Xie, Newberry et al. 2002). Acute phase effects of endotoxemia include hypothermia followed by hyperthermia, hypotension, decreased circulating heterophils associated with increased apoptosis and sequestration in the lung, and increased inflammatory mediators, TL1A, IL‐1β, and IL‐6 (De Boever, Croubels et al. 2009). Increasing amounts of endotoxin in the circulation causes decreased feed consumption and efficiency, decreased body weight and breast meat yield, decreased tibial bone size, weight, calcium content, and breaking strength, and increased mortality, liver weight, plasma ionized calcium, and antibody responses (Mireles, Kim et al. 2005).
Vascular permeability increases leading to the accumulation of fluid and protein in the tissues. Serous membranes become wet and edematous and liquid begins to accumulate in body cavities. Chemotactic factors attract heterophils, which marginate in postcapillary venules and emigrate into surrounding tissues. Between 6 and 12 hours, soft, gelatinous exudate becomes grossly visible. Heterophils can kill E. coli extracellularly by substances such as ß‐defensins released as they degranulate and die (Harmon 1998), (Sugiarto and Yu 2006). After 12 hours there is a progressive shift in inflammatory cells from heterophils to macrophages and lymphocytes. Exudate continues to accumulate and eventually undergoes caseation to form a firm, dry, yellow, irregular, cheese‐like mass. Microscopically, caseous exudate consists of heterophilic granulomatous exudate containing variable numbers of embedded bacterial colonies. A palisade of multinucleated giant cells and macrophages surrounds the exudate (Cheville and Arp 1978). Depending on the size of the mass of exudate, an extended period of time will be required for the exudate to be slowly eroded away by the action of the surrounding phagocytic cells. Viable bacteria persist as microcolonies within the exudate. Epithelial tissue may be restored if damage has not been too severe, but usually there is some degree of fibrosis, which may be complete (scarring) if tissue destruction has been extensive. Exudate containing fibrin undergoes organization and is eventually converted to scar tissue. Gross lesions are inversely related to virulence. Highly virulent strains cause mortality so quickly that gross lesions have little time to develop, whereas birds infected with less virulent strains survive longer and develop more extensive lesions. Infections with APEC serve as a model for studying the molecular aspects of host–pathogen interactions of ExPEC infections. Both pulmonary and systemic infection models have been established using a serogroup O2 strain (Antao, Glodde et al. 2008).
6. Clinical signs and pathology
6.1 Clinical signs
Clinical signs vary from inapparent to total unresponsiveness just prior to death depending on the specific type of disease produced by E. coli. Localized infections generally result in fewer and milder clinical signs than systemic diseases. Coliform cellulitis is typically not detected until the birds are processed. Lameness and retarded growth are seen in birds with skeletal lesions that develop as a sequel to sepsis. Affected birds are typically undersized for the flock and found at the ends of the house, along the side walls, or under feeders or waterers. They may be victims of persecution (“cannibalism”) by other birds. When joints or bones of 1 leg are affected, birds walk with a characteristic hopping motion to keep weight off the affected leg. Birds with lesions in both legs are either nonambulatory or have great difficulty in standing and walking. When the thoracolumbar spine is affected, the birds have an arched back, sit on their hocks, and bear little or no weight on their feet. Occasionally they will sit back on their tail and hocks with their feet elevated off the ground. Birds with chronic lameness have caking of droppings around the vent and on abdominal feathers. Feces are green with white to yellow urates because of anorexia and dehydration. Young birds with omphalitis and infected yolk sacs also may have difficulty in walking because of abdominal distention, which alters weight distribution and impairs balance (McMullin 2020).
Birds with colisepticemia are often terminally moribund or very lethargic. Decreased water consumption is associated with a poor prognosis. Severely affected individual birds are unresponsive when approached, do not react to stimuli, and are easily caught and handled. They sit with their eyes closed in a hunched position with drooping of the head, neck, and wings. The beak may be inserted into the litter to support the head. Dehydration is indicated by dark dry skin, which is especially noticeable in the shanks and feet. Dehydrated young chicks typically have prominent raised folds of skin along the medial and lateral sides of the shanks and toenails that appear black. Although, technically, death is not a clinical sign, this may be the main indication of an outbreak of colibacillosis in a flock. Clinical signs of predisposing or compounding factors often are seen concurrently with signs of E. coli infections (McMullin 2020).
6.2 Pathology
Several localized and systemic types of colibacillosis affect poultry. Often the name is based on the tissue(s) affected or disease process. APEC responsible for different forms of colibacillosis (septicemia, omphalitis, swollen head syndrome) form subpathotypes with different pathogenic, genotypic, and phenotypic traits.
Localized forms of colibacillosis
Coliform omphalitis/yolk sac infection. Omphalitis is an inflammation of the navel (umbilicus). In birds the yolk sac is also usually involved (yolksacculitis) because of its close anatomic relationship to the umbilicus. Infection follows contamination of the unhealed navel with APEC. Fecal contamination of the egg shell and unsanitary conditions in the hatchery are considered the most important sources of infection. Bacteria may be acquired in ovo if the hen has oophoritis or salpingitis or via contamination following artificial insemination. Yolksacculitis also can result from translocation of bacteria from the chick’s intestine or from the bloodstream. In these cases the navel is not affected. Similarly, peritonitis can occur without involvement of the umbilicus.
It is common to recover low numbers of E. coli from normal yolk sacs. Between 0.5% and 6% of eggs from normal hens contain E. coli. Experimentally inoculated hens may shed E. coli in up to 26% of their eggs. Pathogenic strains accounted for 43 of 245 isolates from dead embryos. About 70% of chicks with “mushy chick disease” had E. coli in their yolk sacs. Other types of bacteria also can cause omphalitis, although E. coli is most common. E. coli and Enterococcus faecalis infections accounted for approximately half of the mortality that occurred in layer chicks during the first week after hatching. First week mortality was significantly correlated with total mortality in the flock but not flock uniformity. A variety of E. coli genotypes indicated different sources of infection. For good flock performance, first week mortality needs to be less than 1%. Adhesin factors characterizing omphalitis isolates of E. coli include type 1 (F) fimbriae in 96%, P fimbriae in 8%, and afimbrial adhesins in 16%. Afimbrial adhesin occurred more frequently in omphalitis isolates compared with isolates from cases of salpingitis, swollen head syndrome, or respiratory disease. When genotyped, omphalitis isolates tended to be more similar to commensal isolates than they were to isolates from swollen head syndrome or septicemia. A high percentage of E. coli isolates from eggs, dead embryos, and chicks that died between placement and 7 days of age possessed the virulence genes ipaH (invasion and persistence in cells), eae (attaching and effacing lesions), and cdt (cell distension and death) compared with other APEC (McMullin 2020).
Some embryos may die before hatching, particularly late in incubation; whereas others die at or shortly after hatching. Surviving infected chicks can be a source of E. coli for other chicks in the same hatch. The incidence of birds with omphalitis increases after hatching and declines after about 6 days with occasional losses continuing up to 3 weeks. As few as 10 organisms of serotype O1a:K1:H7 caused 100% mortality in day‐old chicks following yolk sac injection. When birds become infected with low virulent strains there may be no embryo or chick mortality or some may survive although hatchability, chick livability, and relative yolk weights may be affected; the only pathologic finding is retention of infected yolk sacs containing caseated yolk.
Swelling, edema, redness, and possibly small abscesses characterize acute inflammation of the navel. The abdomen is often distended and blood vessels are hyperemic (Figures 5A, 5B). In severe cases, the body wall and overlying skin undergo lysis and are wet and dirty leading to the term “mushy” chicks or poults. Other nonspecific changes such as dehydration, visceral gout, emaciation, vent pasting, and enlarged gall bladder may be seen. The yolk sac is typically distended because yolk has not been absorbed and inflammatory products have been added. Yolk is abnormal in color, consistency, and smell, and may contain visible exudate. Blood vessels of the yolk sac are often prominent. Chicks or poults with infected yolk sacs that live more than 4 days also may have peritonitis, pericarditis, or perihepatitis, indicating local and systemic spread of the organism from the yolk sac.
Microscopically the wall of the infected yolk sac is edematous with mild inflammation. There is an outer connective tissue zone adjacent to a layer of inflammatory cells containing heterophils and macrophages, a layer of giant cells, a zone of necrotic heterophils and masses of bacteria, and then the inner, abnormal yolk contents. A few plasma cells may be found in some yolk sacs.
Consequences of yolk sac infection include deprivation of nutrients and maternal antibodies, absorption of toxins, and spread of E. coli by extension into the body cavity (peritonitis) or systemically to produce colisepticemia and its sequelae (polyserositis, arthritis). Survivors are usually stunted and do poorly. Birds that survive the acute infection have small, firm, persistent yolk sacs (often referred to as “retained” yolk sacs) that contain inspissated exudate and yolk material. E. coli persists in these chronically inflamed yolk sacs and can be isolated from them for weeks to months after hatching. Adhesions to intestines, especially the tip of the duodenal loop, or other visceral organs are common. Rarely the elongated stalk of the yolk sac will wind around the intestine and cause strangulation (McMullin 2020).
Coliform cellulitis (avian cellulitis, inflammatory process, infectious process). Coliform cellulitis is characterized by sheets of serosanguineous to caseated, fibrinoheterophilic exudate in subcutaneous tissues. Lesions, often referred to as “plaques,” are located in the skin over the abdomen or between the thigh and midline. Other colibacillosis lesions, or reduced productivity, occasionally accompany coliform cellulitis, but usually lesions are discovered at processing when inspectors open the thickened yellow abdominal body wall of an otherwise normal carcass.
E. coli are the most frequently isolated organisms from cellulitis lesions. Other bacteria that have been isolated from cellulitis lesions include Pseudomonas aeruginosa, Proteus vulgaris, Enterobacter agglomerans, Pasteurella multocida, Streptococcus dysgalactiae, Aeromonas spp., Staphylococcus aureus, Actinomyces pyogenes, etc., but they are not considered significant (McMullin 2020).
Cellulitis isolates of E. coli belong to the same serogroups as those that cause other forms of colibacillosis. They usually produce colicin and aerobactin. Virulence properties and molecular characteristics are similar among isolates from cellulitis and colisepticemia lesions and normal birds. However, isolates from cellulitis lesions have a greater ability to produce cellulitis in experimentally exposed birds than E. coli isolates from airsacculitis lesions or feces of healthy chickens. By looking for significant associations between the presence of virulence‐associated genes and the cellulitis pathogenicity, it was found that the presence of genes for invasins ibeA and gimB and group II capsule KpsMTII resulted in increased ability of APEC to cause cellulitis.
A vacuolating cytotoxin produced by cellulitis E. coli isolates is also produced by isolates from chickens with colisepticemia and swollen head syndrome but not by isolates from healthy chickens. The cytotoxin is similar to one produced by Helicobacter pylori, except that H. pylori cytotoxin is specific for mammalian cells whereas the avian E. coli cytotoxin is specific for avian cells. Initially, isolates of E. coli from litter and lesions could not be differentiated based on biotyping, suggesting that litter was the source of E. coli in cellulitis lesions. However, genotyping has shown that the prevalence of pathogenic E. coli in a broiler house is independent of the prevalence of other E. coli in the environment. DNA fingerprinting identified the presence of endemic populations of specific cellulitis‐associated E. coli existing in the broiler house environment. These organisms persist for at least 6 months, irrespective of partial or complete cleaning and disinfection as performed in the field, and cause coliform cellulitis in successive flocks (McMullin 2020).
Regional differences in the prevalence of coliform cellulitis emphasize the important roles of environmental and management factors in occurrence of the disease. Increased condemnation rates caused by coliform cellulitis during the past 25 years indicate that changes have occurred in either the occurrence or characteristics of risk factors associated with coliform cellulitis. The most notable change during this time has been in the genotype and phenotype of the bird being raised, so it is not surprising that bird‐related factors contribute significantly to the increased incidence of scratches and subsequent coliform cellulitis.
Fast‐growing, heavy broiler strains are more likely to have an increased prevalence and severity of skin scratches, which predispose to coliform cellulitis (Figure 7). Several reasons may explain this association. The strength of the skin in broilers is related to genetics. The lack of association between scratches and abdominal circumference suggests that strain of bird per se could be a better predictor than body characteristics. Aggressiveness or nervousness of chickens may also be strain dependent. Birds from a more nervous strain could be more active, increasing the chances of being injured or scratched. If aggressiveness is a problem, the source could be farm dependent (e.g., behavioral studies have demonstrated the importance of socialization of the flock by the grower on the birds’ behavior). Rapid growth by modern broiler breeds results in a higher stocking density sooner in the life of the flock, at a time when feathering is not well developed. Poor feathering and crowded conditions could have a significant impact on the incidence of coliform cellulitis. The major histocompatibility complex (MHC) affects the likelihood of an individual chicken developing cellulitis, although not the severity of the lesion. Commercial broilers with MHC type B21 are more susceptible to cellulitis than ones with MHC type B13. However, the severity of lesions was not related to their MHC (McMullin 2020).
Feather cover helps to protect the skin from damage. A positive association exists between scratches and poor feathering. Although little is known about nutritional and environmental factors that affect feather growth and development, birds kept in warm temperatures tend to feather less rapidly than birds kept in cooler temperatures.
Coliform cellulitis occurs more frequently in males than females. The gene responsible for sexing regulates feather growth. Slower feathering males may be more vulnerable to skin injuries because of greater exposure of the skin to potential physical damage. Sex may also contribute to coliform cellulitis because of its association with weight, aggressiveness, or management practices. In addition, production time is longer for roasters than for broilers.
Stocking density plays a dual role as a risk factor. It leads to an increase in skin scratches and stress, but it also contributes by increasing the level of contact between birds. Cellulitis lesions occurred more readily when birds were palpated daily to simulate close contact among birds (McMullin 2020).
Flocks grown on straw were 2.8 times more likely to experience coliform cellulitis than flocks grown on shavings. Physically, straw consists of sharp, pointed pieces that may inflict minor injuries to the skin. Straw may also provide a good medium for growth and multiplication of E. coli because of its ability to hold more moisture than shavings. Similarly, in Brazil, occurrence of coliform cellulitis was greatest in broilers on Brachiaria grass litter compared with corncob, rice shells, or saw dust litter. A positive association also exists between the number of flocks raised on the same litter and cellulitis. However, this association could not be explained by an increase in litter bacterial load.
Furthermore, litter environmental variables (water activity, pH, moisture content, and ammonia levels), as measured in this study, were also not significant. Nevertheless, litter quality should be considered an important factor by those working on reducing this condition in the field. Total down time is negatively associated with coliform cellulitis (i.e., the longer the down time, the lower the incidence of the disease). This supports the hypothesis that the bacterial load in the environment is associated with disease prevalence.
In a prospective study, a positive association between ambient temperature during early grow‐out and cellulitis was found. The predictive model, after controlling for other significant variables, indicated a 40%– 60 % increase in cellulitis as temperatures increased over a range of approximately 15.5°C from–1.7°C to 34.4°C. Low cellulitis prevalence flocks would increase from 0.5% to 0.8 %, whereas high prevalence flocks would increase from 1.2% to 1.9 %. Similar to ambient temperature, increased relative humidity at mid‐grow‐out correlated with increased occurrence of cellulitis. An increase in relative humidity from 36% to 93 % was predicted to increase cellulitis from 0.3% to 0.9 % in low‐prevalence flocks and from 1.0% to 1.9 % in high‐prevalence flocks.
A positive association was observed between coliform cellulitis and feed company in a prospective study. The effect of nutrition on the pathogenesis of the disease is not well known. Amino acid levels in the feed may be important. Feed deficient in cysteine and methionine can cause nervousness and affect feathering. A relative deficiency occurs in feeds with high energy to total protein ratios. High‐energy feeds may also contribute to coliform cellulitis by increasing fat deposition in the skin, which may result in the skin being more susceptible to scratches and injuries (McMullin 2020).
The occurrence of coliform cellulitis was higher in vegetarian broilers compared with broilers fed feeds containing animal products. Condemnation rates for birds fed a standard diet, which contained growth promotants, antibiotics, and anticoccidials, was substantially lower (0.26%) than for birds fed a vegetarian or organic feed without additives (1.18%).
Older chickens are more likely to develop lesions of cellulitis following inoculation of scratches or subcutaneous injection than young chickens, which tend todevelop systemic disease and experience high mortality. Cellulitis has also been described in quail. In turkeys, cellulitis is similar to gangrenous dermatitis and differs from coliform cellulitis in chickens.
Cellulitis lesions are primarily unilateral and located on the abdomen or thigh. Skin color varies from normal to yellow or red‐brown, and the skin may be swollen at the site of inflammation (Figure 7). The size of the lesion normally varies between 1–10 cm. Scratches and scabs on the skin overlying the lesions often can be identified (Figure 8). Beneath the skin, there is subcutaneous edema, exudate, and muscle hemorrhage. A fibrinous to caseous plaque between the muscle and subcutis is the characteristic lesion (Figure 6H).
Lesions develop rapidly; exudate is visible as early as 6 hours postinfection, and the caseous plaque could be experimentally produced within 18–24 hours postinfection. Rapid lesion development suggests that events occurring late in the life of the flock could be important in the development of lesions found at processing. When birds were inoculated experimentally with E. coli strains isolated from coliform cellulitis lesions, the highest percentage of birds developing typical lesions had been challenged only 3 days prior to processing. Lesions were still present 3 weeks postinoculation (McMullin 2020).
Experimental exposure of young chickens to cellulitis isolates of E. coli results in septicemia, death, or marked stunting, indicating that most birds affected by E. coli in the hatchery would either die or be culled before reaching the processing plant. No association between cellulitis and the source of eggs, age of parent flocks, total bacterial count, and coliform count in the hatchers was found.
Skin trauma, especially scratches, provides the main portal of entry into the host for specific cellulitis‐type E. coli present in the litter. Applying bacteria to feather follicles from which the feather had been pulled did not cause coliform cellulitis. Oral feeding or swabbing the navel of young chickens did not produce cellulitis but did result in mortality, depressed growth, and other types of colibacillosis, which was dose dependent. The disease is reproduced readily by swabbing damaged skin with broth cultures or subcutaneous inoculation (McMullin 2020).
When a fast‐growing strain of broiler chicken was compared with a strain of leghorns, the broiler strain was more predisposed to cellulitis because of an inferior first line of defense of their skin. In the broilers, wound‐healing was slower, lesions were more severe and covered a larger area, and mobilization and functionality of phagocytic cells were inferior. Usually, an affected bird has only skin lesions, but concurrent lesions of systemic colibacillosis occasionally can be found suggesting that cellulitis may result from systemic spread or, conversely, that localized lesions in the skin can be a source for systemic disease. The latter is inversely correlated with age (i.e., the younger the bird, the more likely it is to develop systemic disease). Lesions have been correlated with other categories of condemnation in which E. coli would be expected to play a significant role (septicemia, airsacculitis, etc.).
A positive association between cellulitis and ascites has been shown. Ascites is a common condition in broiler chickens characterized by an abnormally large abdomen. Because most cellulitis lesions are located in the abdominal area, it may be that ascites is a biological predisposing factor for cellulitis. It also is possible that both conditions may share common risk factors such as rapid growth.
Valgus‐varus leg deformity, characterized by lateral or medial deviation of the distal tibiotarsus with a corresponding deviation of the tarsometatarsus, occurred more frequently in carcasses condemned for cellulitis. Valgus‐varus deformity is considered to be the most frequent cause of leg weakness and lameness in broiler chickens. However, the association between valgus‐varus deformity and coliform cellulitis needs to be interpreted with caution because of potential confounding with sex and breed. Most valgus‐varus deformity affects male birds, and the incidence of coliform cellulitis can vary with breed. Birds with valgus‐varus leg deformity spend more time lying on the floor, which results in greater contact exposure between the skin and the E. coli present in the litter. Also, prolonged resting by lame birds may result in skin damage as other birds tread on them (McMullin 2020).
Cellulitis lesions are identified readily at processing, normally making it possible to use condemnation results to assess control strategies. However, an epidemiological study in Ontario has found that 30% of the variation incellulitis prevalence was dependent on the slaughter plant. Therefore, the possibility of misclassification may exist, and should be considered in an investigation. Lesions should be cultured aseptically to determine the presence of E. coli.
There is no treatment for coliform cellulitis, and eradication of the disease will not be possible because of the ubiquitous occurrence of E. coli. Advances in the development of immunoprotective agents or immu-nomodulators suggest that a molecular approach to cellulitis control is possible, although not currently practical or economical. However, by carefully managing the environment and nutrition of the modern, fast‐growing, heavy broiler, it is possible to reduce substantially the incidence and impact of the disease. A key aspect of any control strategy is its cost–benefit. Adequate monitoring to ensure implementation and compliance of control strategies will be needed to determine their effectiveness. The following are some recommendations.
Very early lesions consist mainly of serosanguineous fluid in contrast to the caseous lesions observed after 24 hours postinfection. A high prevalence of acute lesions indicates that events occurring just prior to or during transportation should be investigated, especially if at least 10 hours exists between load‐out and processing. In contrast, a majority of chronic lesions would indicate the need to focus on earlier events that occurred during the grow‐out period.
Problem flocks should be compared with flocks that did well during the same time period within the same company and the risk factors determined for each type of flock. Any management or environmental factors that affect the birds’ resistance or contribute to skin scratches should be identified. Pay special attention to stocking density, feeder and waterer space (effective space, i.e., in some houses, the space is available, but the drinkers or feeders are not all functional), migration fencing, type of litter, quality of litter, and feed restriction and lighting programs. Any intervention must first focus on improving the environment of the birds. This includes good sanitation to reduce the bacterial load of the environment (McMullin 2020).
Swollen head syndrome. Swollen head syndrome (SHS) is an acute to subacute cellulitis involving the periorbital and adjacent subcutaneous tissues of the head. SHS was first described in broilers in South Africa associated with E. coli and an unidentified coronavirus infection. The disease has subsequently been described in most intense poultry‐producing areas of the world. The disease also affects turkeys and guinea fowl.
Swelling of the head is caused by inflammatory exudate beneath the skin that accumulates in response to bacteria, usually E. coli, following upper respiratory viral infections (e.g., avian metapneumovirus, infectious bronchitis virus). Ammonia aggravates the disease. The portal of entry is considered to be the conjunctiva or inflamed mucous membranes of the sinuses or nasal cavity. Possible infection via the Eustachian tube also has been suggested. Microscopic lesions include fibrinoheterophilic inflammation and heterophilic granulomas in the air spaces of the cranial bones, middle ear, and facial skin. Lymphoplasmacytic conjunctivitis and tracheitis with formation of germinal centers have also been observed.
Although the pathogenesis of SHS has not been established, conjunctival‐associated lymphoid tissue inflamed from virus infection and/or ammonia irritation may serve as the site through which bacteria gain access to subcutaneous tissues. Periorbital inflammation is typically seen early in the disease and hyperplastic lymphoid tissue has been shown to be a site where E. coli penetrates mucosal surfaces. Scarifying the conjunctival mucosa and instilling a pure culture of E. coli, or inoculation of E. coli into submucosal or subcutaneous tissues, will reproduce the disease. Intranasal inoculation of avian metapneumovirus and E. coli failed to reproduce the disease. Swollen head syndrome did not occur when day‐old chicks were inoculated supraconjunctivally with either avian metapneumovirus or E. coli, but they did develop clinical disease, which was most severe when the chicks received both agents (McMullin 2020).
E. coli isolates from SHS cases possess several virulence factors including fimbrial adhesins, colicin production, aerobactin, and complement resistance. In general, SHS isolates have virulence attributes similar to isolates from cases of septicemia, except colicin production and iron‐acquisition siderophores were more frequent in SHS isolates. The colicins produced by SHS strains often differed from ColV. The majority of strains were motile, but presence of K1 capsule was infrequent. Similar results were found in a subsequent study in which SHS isolates were more similar to isolates that cause septicemia than isolates that cause omphalitis or commensal isolates except type 1 and curli fimbriae, and temperature‐sensitive hemagglutinin (tsh) were more frequent in SHS isolates. A transferable 60 MDa plasmid from an SHS E. coli isolate contained genes for cell adhesion, colicin production, and tsh.
Adhesin factors occurring in SHS E. coli isolates included type 1 in 94% and P fimbriae in 28%. The P‐fimbrial adhesin factor occurred more frequently in SHS isolates compared with isolates from cases of salpingitis, omphalitis, or respiratory disease. A unique Shiga toxin (VT2y) that may be involved in the pathogenesis of SHS was identified in a high percentage of SHS E. coli isolates. Additionally another toxin, similar to one produced by Bacillus cereus that is highly lethal for mice following injection, was identified in SHS isolates.Other work showed that flgE (flagellar hook), tyrR (transcriptional regulator), potF (putrescine transporter), yehD (putative adhesin) and bfr (bacterioferritin) were attenuated compared with a wild‐type strain in a 1‐day‐ old chickens infection model (McMullin 2020).
Diarrheal disease. Primary enteritis is a common manifestation of E. coli infections in mammals including humans, but is considered rare in poultry. Diarrhea results from infections with enterotoxigenic (ETEC), enterohemorrhagic (EHEC), enteropathogenic (EPEC), or enteroinvasive E. coli (EIEC); each type possessing certain virulence factors that determine the characteristics for each type of enteric disease. EHEC and EPEC strains produce attaching and effacing lesions on intestinal mucosal surfaces. Collectively, these strains are called attaching and effacing E. coli (AEEC). Similar lesions are also produced by the closely related species, E. albertii. Intestinal E. coli in poultry have been poorly studied, except as commensal strains for comparison with APEC or reservoirs of virulence genes that occur in human strains, so our knowledge of the role that E. coli may play in intestinal disease is limited.
Enterotoxigenicity caused by ETEC strains is uncommon in APEC. Most surveys for heat‐stable and heat‐labile enterotoxins either fail to find any positive isolates or identify only a few. ETEC that elaborated toxins capable of causing fluid accumulation in ligated intestinal loops of chickens were recovered from chickens with diarrhea, and an O15 APEC strain that produced heat‐labile toxin II was isolated from ostrich chicks experiencing severe diarrhea and high mortality.
Natural and experimental infections with AEEC or presence of eae gene have been reported in chickens, turkeys, pigeons, ducks, psittacines, and other avian species. Infections with infectious bursal disease (IBD) virus in chickens and adenovirus infection in the pigeon were considered possible predisposing factors to AEEC infection. In turkey poults, coinfection of EPEC and turkey coronavirus (TCV) resulted in severe stunting and very high mortality. Clinical disease was most severe when poults were infected with TCV prior to inoculation with EPEC. Ten of 12 commercial turkey flocks experiencing high mortality because of poult enteritis mortality syndrome (PEMS) were infected with EPEC, confirming the importance of natural EPEC infection as a cause of mortality in young turkeys. Infections with IBD virus in chickens and adenovirus infection in the pigeon were considered possible predisposing factors to AEEC infection. In turkey poults, coinfection of EPEC and TCV resulted in severe stunting and very high mortality (McMullin 2020).
Birds infected with AEEC may be clinically normal or have diarrhea and be dehydrated. In clinically affected birds, the intestines are pale and distended with fluid, which may contain visible flecks of mucus and exudate. Ceca are often the most obviously affected part of the digestive tract. They are typically distended with pale brown fluid and gas. Bacteria intimately attach to the surface of enterocytes causing effacement of microvilli, pitting, and pedestal formation, which are best seen by electron microscopy (Figure 9). Lesions are most common in the ceca. Organisms are readily identified in tissue sections using Giemsa stain or by immunohisto chemical methods.
Experiments to define a role for E. coli in malabsorption syndrome of chickens have not been successful. In contrast, specific strains of E. coli have been associated with PEMS. Turkey astrovirus, an agent involved in PEMS, impairs macrophage function, which could explain the enhanced susceptibility of affected poults to secondary bacterial infections such as colibacillosis.
Diseases resulting from infection with EIEC have not been described but are likely, especially in the case of neonatal septicemia. EIEC possess genes such as ipa, which encodes a virulence factor that provides the organism with the ability to penetrate and survive within cells. The most frequently identified virulence gene in E. coli isolated from eggs, dead embryos, and chicks with omphalitis/yolk sac infections was ipaH. Most of the ipaH+ isolates (62 of 80; 77.5%) came from liver or yolk sac of chicks that died between 3 and 7 days of age, which corresponded to a period of increased mortality. Further characterization of the ipaH+ isolates revealed properties that did not match those of typical EIEC and the existence of specific EIEC clone complexes among avian isolates. Cell invasion was confirmed in vitro (McMullin 2020).
Venereal colibacillosis (acute vaginitis). Venereal colibacillosis is an acute and frequently fatal vaginitis that affects turkey breeder hens shortly after they are first inseminated usually in the first weeks of egg production. Puncturing the hymen of young turkey hens can lead to a severe localized E. coli infection characterized by vaginitis, cloacal and intestinal prolapse, peritonitis, egg binding, and internal laying. The affected mucosa is markedly thickened, ulcerated, and covered with a diphtheritic, caseonecrotic membrane, which causes obstruction of the lower reproductive tract. The thickness of these membranes posed an obstruction to egg passage leading to internal laying and egg peritonitis. The upper oviduct is grossly and histologically normal. Flocks can have losses of up to 8% because of increased mortality and culling. Egg production is decreased and there is an increased number of cull eggs because of small size. Swabs from cloaca and vagina produced numerous colonies of only E. coli. No other infectious agents have been identified as contributing to the disease. Anecdotal reports indicate a similar disease can affect young broiler breeders as they come into production (McMullin 2020).
Coliform salpingitis/peritonitis/salpingoperitonitis (adult). Inflammation of the oviduct caused by E. coli results in decreased egg production and sporadic mortality. It is one of the most common causes of mortality in commercial layer and breeder chickens and also affects other female birds, especially ducks, geese, and quail. Accumulations of caseating exudate in the body cavity resemble coagulated yolk, which is the reason for the common name “egg peritonitis.” Yolk peritonitis is a mild to moderate diffuse peritonitis without exudate resembling coagulated yolk that results from free yolk in the body cavity. Yolk peritonitis is usually associated with bursting atresia that occurs during acute ovarian regression. Marked exudation, extensive inflammation, and positive cultures characterize coliform peritonitis and serve to distinguish it from yolk peritonitis.
Salpingitis and egg binding may occur concurrently, which can cause confusion because both result in an obstructive mass within the oviduct. If an egg is not grossly visible, cutting through the mass in the oviduct and finding a shelled egg in the center indicates egg binding.
Infection occurs when E. coli ascends the oviduct from the cloaca. Injecting large (109) numbers of bacteria into the reproductive tract reproduces the disease. Mucosal infections with viruses (e.g., infectious bronchitis virus) or mycoplasmas also may predispose a bird to salpingitis. M. synoviae infection increased the occurrence of coliform peritonitis. Coinfection with E. coli and Tetratrichomonas occurred in Pekin duck breeders with salpingitis. Heavy egg production and associated estrogenic activity predispose hens to salpingitis by relaxing the sphincter between the vagina and cloaca. Spread of E. coli to the oviduct from an airsacculitis is also possible, but this form of salpingitis occurs more frequently in young birds as part of a systemic infection.
Isolates of APEC from birds with salpingitis have similar virulence characteristics to those that cause airsacculitis. In a study of 30 isolates, 11 belonged to serogroups O2 and O78 whereas 10 were untypeable. Twenty‐seven of the isolates were of either high or intermediate virulence in a day‐old chick assay. Most isolates possessed type 1 fimbriae and adhered to oviductal epithelium, especially from adult breeders, and they had the ability to acquire iron when grown in iron‐deficient medium. Isolates were resistant to serum from young breeders, but sensitive to serum from older breeders. In a separate study, type 1 fimbriae also were identified in 49 of 50 isolates from broiler breeders with salpingitis; few isolates possessed other fimbrial types. Presence of type 1 fimbriae can be considered a characteristic of APEC salpingitis isolates.
E. coli was isolated from the cloacae of clinically normal birds and dead birds with peritonitis, and from the oviducts and peritoneal cavities of birds with peritonitis, but not from these sites in clinically normal birds. E. coli from the oviducts and peritoneal cavities of each of the dead birds had identical characteristics. Isolates from one flock belonged to serogroup O78, phyologentic group A, and contained virulence genes iroN, sitA, iutA, tsh, and iss. Isolates from a second flock belonged to serogroup 111, phylogenetic group D, and contained virulence genes iroN, sitA, iutA, traT, iss, and ompT. The similarity among isolates from hens in each flock indicated a single clone of E. coli affected that specific flock. Molecular characteristics of E. coli isolated from layers with salpingitis, peritonitis, and salpingoperitonitis also were clonal and possessed several of the same virulence factors including iss, sfa, tsh, iucC, ibeA, and sitA. Specific clones caused colibacillosis in free‐range layer flocks indicating they were primary pathogens that did not require predisposing factors to cause disease (McMullin 2020).
In chronic cases, the oviduct is markedly distended and thin‐walled with single or multiple masses of caseous exudate in the general form of the oviduct (Figure 6A). The mass of exudate may expand to the point that it fills most of the body cavity. Rupture of the oviduct is possible. Exudate is laminated, often contains a central egg, shells, and/or membranes, and is malodorous. Spread of the organism into the body cavity through the compromised oviduct wall or the open end of the infundibulum leads to concurrent peritonitis, which is termed salpingoperitonitis when there is involvement of both the oviduct and peritoneum. Peritonitis in the absence of salpingitis also can occur but is uncommon. Acute cases have less exudate in the oviduct or peritoneum that tends to be soft and not as caseated (Figure 6B). Affected birds are incapable of laying eggs although they typically continue to ovulate. Repeated ovulations and albumen secretion are responsible for the laminated appearance of the oviductal masses. Abdominal laying and misovulated ova may accompany salpingitis and contribute to peritonitis.
Microscopically, the tissue reaction in the oviduct is surprisingly mild in view of the marked gross lesions. It primarily consists of multifocal to diffuse heterophil accumulations subjacent to the epithelium and caseous exudate in the lumen, which often contains bacterial colonies. Lymphoid foci develop in the mucosa with time and indicate chronicity (McMullin 2020).
Coliform orchitis/epididymitis/epididymo‐orchitis. An ascending E. coli infection of the male reproductive tract, analogous to that resulting in salpingitis in the hen, occurs infrequently in roosters. Testicles are swollen, firm, inflamed, irregularly shaped, and have a mosaic of necrotic and viable tissue when opened. Heavy growth of E. coli can be obtained from the testicle and epididymis (McMullin 2020).
Systemic forms of colibacillosis
Colisepticemia. Presence of virulent E. coli in the blood stream defines colisepticemia. Virulence and number of organisms balanced against efficacy of host defenses determine duration, degree, and outcome of the disease, as well as the pattern and severity of lesions. Colisepticemia progresses through the following stages: acute septicemia, subacute polyserositis, and chronic granulomatous inflammation. Whereas lesions are typical of colisepticemia, other bacteria capable of producing septicemia also can cause similar changes. Characteristic features of colisepticemia at necropsy are tissues that develop a green discoloration following exposure to air and a characteristic odor, possibly related to indole produced by the organism. The bursa of Fabricius is often atrophic or inflamed as a result of colisepticemia. It should not be interpreted that a small bursa is evidence of a prior immune‐suppressing disease such as IBD.
Pericarditis is common and is a characteristic of coli-septicemia. It is usually associated with myocarditis, which results in marked changes in the electrocardiogram, often before gross lesions appear. Vessels in the pericardium become increasingly prominent because of hyperemia and the pericardium becomes cloudy and edematous. Initially, fluid and soft masses of pale exudate accumulate within the pericardial sac followed by fibrinous exudate (Figure 5C). Exudate can be seen loosely adhering to the epicardium when the pericardialsac is opened. As the disease progresses, exudate increases, becomes more cellular (fibrinoheterophilic), and undergoes caseation. The pericardial sac adheres to the epicardium with chronicity.
Microscopically, the same progression of lesion development is seen. Serous and serofibrinous exudate is seen initially followed by increasing numbers of heterophils and subsequently macrophages. Within the myocardium, particularly close to the epicardium, there are accumulations of lymphoid cells and by 7–10 days there also are many plasma cells. Subsequently, exudate in the pericardial sac undergoes organization (Figure 5G), which, in survivors, eventually results in constrictive pericarditis and liver fibrosis caused by chronic passive congestion. Cardiac lesions reduce arterial blood pressure from a norm of about 150mmHg to about 40mmHg just before death.
Several distinct clinical forms of colisepticemia can be distinguished depending on how the organism gains access to the circulation and the age and type of bird (McMullin 2020).
Respiratory‐origin colisepticemia. Respiratory‐origin colisepticemia affects both chickens and turkeys and is the most common type of colisepticemia. E. coli gains access to the circulation following damage to the respiratory mucosa by infectious or noninfectious agents. IBV and Newcastle disease virus (NDV), including vaccine strains, mycoplasmas, and ammonia, are the most common predisposing agents. Avian metapneumovirus infection increases susceptibility of turkeys to colisepticemia. Severity of the resulting disease, which is commonly referred to as airsacculitis, CRD, or multicausal respiratory disease, is directly related to the number of agents that are involved. A diversity of E. coli serotypes can be identified in a disease outbreak. Those in the tissues are usually different from those in the intestinal tract of the same bird but can be found in the intestinal tracts of other birds and the environment.
Susceptibility is increased when IBV, IBD or NDV infection occurs. Five days after administration of a vaccine strain of NDV, clearance of aerosol‐administered E. coli is reduced. Microscopically, 3–8 layers of immature, nonciliated cells replace the pseudostratified, columnar epithelium of the trachea. Mixed IBV E. coli infections are more severe than those caused by either agent alone. Antibodies against E. coli produced by chickens infected with IBD virus and/or IBV had a significant decrease in opsonizing ability compared with antibodies produced by normal chickens. Reduced opsonization of the organism resulted in decreased macrophage function, which may help explain the frequent infection with E. coli that follows IBV infection. Mycoplasmal infection increases susceptibility to E. coli about 12–16 days postinoculation, and susceptibility persists for at least 30 days. Coinfection with IBV or NDV in addition to mycoplasma further decreases resistance to E. coli and the period of increased susceptibility begins earlier and persists longer.
Inhaled coliform‐contaminated dust has been implicated as one of the most important sources for infecting air sacs of susceptible birds. Exposure to chicken‐house dust and ammonia results in deciliation of the upper respiratory tract of birds permitting inhaled E. coli to colonize and cause respiratory infection.
Lesions are prominent in respiratory tissues (trachea, lungs, air sacs), pericardial sac, and peritoneal cavities and are typical of the subacute polyserositis stage of colibacillosis (Figures 5C, 5D, 5F). Infected air sacs are thickened and often have caseous exudate on the respiratory surface. Microscopically, the earliest changes consist of edema and heterophil infiltration. Mononuclear phagocytes are frequently seen 12 hours after inoculation. Later, macrophages become common, with giant cells along margins of necrotic areas. There is fibroblast proliferation and accumulation of vast numbers of necrotic heterophils in caseous exudate. Bacterial colonies are often present in caseous exudate and contain numerous organisms. E. coli colonies have a typical appearance in histologic sections. They are usually circular with concentrated bacilli forming a distinct smooth perimeter with fewer bacilli and spaces centrally. They stain negative with tissue Gram stain. Lesions of predis posing respiratory disease are usually present in the trachea and lungs and consist of lymphoid follicles, epithelial hyperplasia, and epithelium‐lined air passages that may contain heterophils. Pneumonia and pleuropneumonia are more common in turkeys whereas chickens usually have pleuritis or pleuropneumonia with less lung involvement. Extension of the disease process into the oviduct from the left abdominal air sac may occur and cause salpingitis in juvenile birds (Figure 5H).
Inoculating pathogenic E. coli or bacteria‐free culture filtrates into the air sac readily reproduces lesions of uncomplicated coliform infection. Airsacculitis occurs within 1.5 hours. Bacteremia and pericarditis develop within 6 hours. In birds that survive, lesions are well‐developed by 48 hours post-inoculation. Most mortality occurs during the first 5 days. Recovery is usually rapid if birds survive the initial infection, although a few with persistent anorexia become emaciated and die. Have developed a defined method for determining susceptibility to colibacillosis that is reproducible and has been used to determine the relative innate (genetic) susceptibility of various broiler chicken genotypes to the disease. Another study observed that preceding infection with IBV vaccine does not impair the clearance of E. coli in the respiratory tract of broilers, but rather induces an exaggerated inflammatory response only in the airsacs (McMullin 2020).
Enteric‐origin colisepticemia. Enteric‐origin colisepticemia is most common in turkeys. E. coli gain access to the circulation and tissues following damage to the intestinal mucosa by infectious agents. The most common predisposing agent is hemorrhagic enteritis virus. Usually only 1 or 2 types of E. coli are involved in the disease outbreak, and those in the tissues and intestinal tract of each bird tend to be the same.
Lesions are typical of the acute septicemic stage of colibacillosis. Affected birds are in good physical condition and often have full crops containing feed and water. The most characteristic lesions are congestion or green discoloration of the liver, marked enlargement and congestion of the spleen, and congested muscles (Figure 6C). Microscopically the spleen is congested with proteinaceous fluid in sinuses and has multifocal necrosis, often containing intralesional bacteria. Fibrin thrombi are present in liver sinusoids and occasionally renal glomeruli. In some cases, multiple, pale foci in the liver are seen. Microscopically these are areas of acute necrosis initially, but with time they evolve into granulomatous hepatitis in survivors (Figure 6D). After a few days birds eventually develop lesions similar to those of respiratory‐origin colisepticemia.
Hemorrhagic septicemia. This form of colibacillosis occurs in turkeys and is characterized by generalized circulatory disturbances, discoloration of subserosal fat, bloody fluid on serosal surfaces, pulmonary edema and hemorrhage, and enlargement of the liver, spleen, and kidneys. Generalized necrosis of the liver and multifocal necrosis of the spleen are seen on cut surfaces. Pure cultures of E. coli are obtained from the liver, spleen, and pericardium. Characterization of isolates from 7 affected flocks showed they belonged to multiple serogroups (O1, O2, O18, O78, and O111). For each flock, isolates were clonal based on serotype, plasmid profiling, ribotyping, and MLST. Virulence factors were similar among all isolates in spite of differences in serotype and phenotype, and included F11 pili, iucD, iss, vat, tsh, and colicin V (McMullin 2020).
Neonatal colisepticemia. Chicks are affected within the first 24–48 hours after hatching. Mortality remains elevated for 2–3 weeks and usually totals 10%–20%. Up to 5% of the flock may be stunted and require culling. Unaffected birds grow normally and the disease does not appear to spread. Initial lesions consist of congested lungs, edematous serous membranes, and splenomegaly. The proventriculus and lungs develop a dark color that can approach black as the interval between death and necropsy increases. Microscopically, bacteria are numerous in affected tissues and easily identified. After a few days the typical pattern of acute, fibrinoheterophilic polyserositis involving the pericardial sac, pleura, air sacs, and peritoneum becomes evident. Lesions are often extensive and severe in birds that survive into the second week. Occasionally birds with arthritis or osteomyelitis may be found late in the disease. Most affected birds have yolk sac abscesses suggesting the navel is the portal of entry. Alternatively, in ovo infection may be responsible (McMullin 2020).
Layer colisepticemia. Colisepticemia is usually a disease of young birds, but occasional outbreaks of acute E. coli infection resembling fowl typhoid or fowl cholera occurs in mature chickens and turkeys. Acute colibacillosis in layers is being seen with increasing frequency. The majority of outbreaks are associated with onset of egg production, but less frequently they occur at an older age, or may continue as the flock ages and potentially spread to older flocks on the same farm. The disease may reoccur in the same flock or subsequent flocks placed on farms or in houses where affected flocks had been previously. Death usually occurred suddenly without premonitory signs, although depression and/or dirty vents were observed in some affected hens in approximately half of the flocks. Weekly mortality was significantly higher in affected flocks than age‐matched control flocks (0.26%–1.71% vs. 0.07%–0.30%). Cumulative mortality ranged up to 10% and mortality remained elevated for 3–10 weeks. Polyserositis (perihepatitis, pericarditis) and peritonitis associated with free yolk in the peritoneal cavity were present in most birds at necropsy. Oophoritis and salpingitis occurred less frequently (McMullin 2020).
Isolates of E. coli from outbreaks in Italy were lactose negative, nonmotile, and belonged to serogroup O111. Intramuscular inoculation of the O111 APEC reproduced the disease whereas it developed in only a few birds following oro‐nasal administration. In contrast, the majority of isolates from outbreaks in Belgium were serogroup O78. Outbreak isolates were more likely to have P fimbriae (F11), especially if they were serogroup O78 and recovered from the heart. Serogroup O78 isolates also had the lowest percentage of motile strains. A number of virulence factors were significantly more frequent in outbreak isolates compared with control isolates. However, when cecal isolates and extraintestinal isolates within either the outbreak or control groups were compared, they were not significantly different. Collectively no virulence factors or combination of factors were found only in outbreak isolates (McMullin 2020).
The pathogenesis of the disease is unknown, but stress associated with onset of egg production is believed to be an important contributing factor. Ascending infections via the oviduct have been suggested as a means by which E. coli gain access to systemic tissues, but in a recent study, higher colonization rates of the trachea, but not the oviduct, in affected flocks suggests layer colisepticemia may be aerogenous. Lack of recognized stressors or indications of diseases known to predispose chickens to colisepticemia suggest layer colisepticemia results from a primary E. coli infection.
Risk factors for developing layer colisepticemia include close proximity to other poultry farms and higher stocking density. Control has been through chlorination of water or treatment of the flock with appropriate antibiotics.
Coliform septicemia of ducks. Coliform septicemia of ducks is characterized by moist, granular to curd‐like exudate of variable thickness causing pericarditis, perihepatitis, and airsacculitis. A characteristic odor is often noted at necropsy. Liver is frequently swollen, dark, and bile stained, and spleen is swollen and dark. E. coli (usually O78) can be recovered from any internal organ. Riemerella anatipestifer causes similar lesions, but it can be identified by appropriate cultural procedures.
Coliform septicemia occurs throughout the growing season but is most frequent in late fall and winter. All ages of ducklings are susceptible. Distribution of losses suggests individual farms, rather than hatcheries, are the source of infection. Poor husbandry and marked contamination of pond water used by ducklings were contributing factors to an outbreak of colisepticemia in captive mallards (McMullin 2020).
Colisepticemia sequelae. Death is the usual outcome of colisepticemia, but some birds may completely recover or recover with residual sequelae. If the bird does not control E. coli, it can localize in poorly protected (“immunologically privileged”) sites including the brain, eyes, synovial tissues (joints, tendon sheaths, sternal bursa), and bones. In immature females, salpingitis can occur when there is involvement of nearby air sacs. IBV infection of the oviduct may also be an important predisposing factor in juvenile salpingitis. After E. coli is no longer present, constrictive pericarditis develops as exudate in the pericardial sac undergoes organization, and liver becomes fibrotic (Figure 5G). Ascites may develop because of residual pulmonary damage from combined E. coli IBV infection. It also is possible for ascites to develop from the direct action of endotoxin on the pulmonary vascular system. Endotoxin causes vasoconstriction leading to pulmonary hypertension and the potential to develop ascites (pulmonary hypertension syndrome) (McMullin 2020).
Meningitis and meningoencephalitis. E. coli localization in the brain, although uncommon, has been reported. Meninges are affected (meningitis) but in some birds there also is involvement of the brain (encephalitis) and ventricles (ventriculitis). Meningeal lesions are evident at necropsy as areas of discoloration, often adjacent to major blood vessels. Fibrinoheterophilic to heterophilic exudate is seen microscopically early in the infection; the lesion becomes more granulomatous with time. Bacteria are usually numerous within lesions but may not form distinct colonies (McMullin 2020).
Panophthalmitis. As with the brain, involvement of the eye is uncommon. However, if it is infected the resulting panophthalmitis is severe. Typically there is hypopyon and hyphema, and infection is unilateral (Figure 6F). The eye is swollen, cloudy to opaque, and may be hyperemic initially. Later the eye shrinks as it undergoes atrophy. Fibrinoheterophilic exudate and numerous bacterial colonies are present throughout the eye. Inflammation, especially adjacent to necrotic tissue, becomes granulomatous with time. Varying degrees of retinal detachment, retinal atrophy, and lysis of the lens also may be seen. The organism persists in the diseased eye for long periods of time (McMullin 2020).
Osteoarthritis and synovitis. Localization of E. coli in bones and synovial tissues is a common sequel to colisepticemia (Figure 6E). The term osteoarthritis is used when a joint is inflamed and 1 or more bones making up that joint have osteomyelitis. Polyarthritis refers to involvement of more than 1 joint. Bacterial chondronecrosis with osteomyelitis (BCO) is another name that has been used.
Affected birds likely have insufficient resistance to clear bacteria completely. Hematogenous spread of E. coli following hemorrhagic enteritis virus infection of turkeys resulted in synovitis, osteomyelitis, and green liver discoloration. Intravenous inoculation to simulate hematogenous spread to bones and joints has been used to reproduce the lesions, but mortality from the initial septicemia is often high. A preferable method is to inoculate the air sac of birds with low numbers of E. coli after a pretreatment with dexamethasone to immunosuppress the birds.
Mild to severe lameness and poor growth are seen clinically and affected birds are more likely to be victims of persecution (“cannibalism”). Often multiple sites are involved. Bacteria colonize the vascular sprouts that invade the physis of a growing bone provoking an inflammatory response that results in osteomyelitis. Transphyseal blood vessels in birds serve as conduits for the process to spread into the joint and surrounding soft tissues. Compared with clinically normal turkeys, lame turkeys had the following: higher splenic and liver weights, lower body and bursal weights, decreased cellular immunity, normal to increased humoral immunity, decreased circulating lymphocytes, increased circulating total leukocytes, monocytes, and heterophils, normal to marginally depressed phagocyte function, increased serum protein, uric acid, and blood urea nitrogen, and decreased hemoglobin, iron, alkaline phosphatase, and gamma‐glutamyl‐transferase. Bones most often affected are the tibiotarsus, femur, thoracolumbar vertebra, and humerus. Proximal physes of long bones are more frequently affected than distal physes. Lesions typically form where endochondral ossification is occurring and extend proximally to involve the adjacent physeal cartilage. It is common to find both osteomyelitis and tibial dyschondroplasia together, but this is most likely because of their occurrence at the same location rather than a cause and effect relationship. Osteomyelitis is easily recognized on gross examination of bones opened to expose the physes, but small lesions that can only be seen microscopically also occur.
Hock, stifle, hip, and wing joints and articulations of the free thoracic vertebra are sites where arthritis is most likely to occur. Lesions in other joints are less common. Trauma to joints and growing bones may predispose to the development of lesions. Tenosynovitis frequently accompanies arthritis. Less commonly, spread of the inflammatory process from a joint into the periarticular tissues occurs. An infectious sternal bursitis is also common but must be distinguished from traumatic sternal bursitis in which fluid is seen but not exudate. When inflammatory lesions involve the shoulder joint or proximal humerus, extensive exudate can accumulate between the superficial and deep pectoral muscles (Figure 5E). Lesions that develop in joint spaces of the articulating free thoracic vertebra are characterized by spondylitis, which results in progressive paresis and paralysis (Figure 10). Lesions in the distal articulation of the free thoracic vertebra occur more commonly than lesions in its proximal articulation or both articulations (McMullin 2020).
Turkey osteomyelitis complex. Turkeys with turkey osteomyelitis complex (TOC) have infectious, inflammatory lesions in bones, joints, or periarticular soft tissues and enlarged, green discolored livers that are used at processing to indicate the possible presence of intraosseous lesions. Green liver discoloration is rarely identified in the field even in lame turkeys. Most often green discolored livers are detected at processing and result in downgrading and, depending on severity, partial or whole bird condemnation. It is likely green discoloration of the liver is more evident in processed birds because they have bled out in contrast to birds in flocks that either die or are euthanized.
In TOC, osteomyelitis and arthritis occur as described previously. Spondylitis of the articulating free thoracic vertebra can be associated with green liver discoloration. Not all birds with green discolored livers have TOC, but generally birds with TOC have discolored livers. Feed and water withdrawal do not cause green liver discoloration, but infection with M. synoviae can be associated with a high percentage of carcasses with green discolored livers in the absence of TOC. The Food Safety Inspection Service in the United States uses green discoloration of the liver at processing to identify carcasses that may have TOC so that any abnormal tissue can be removed from the food chain. Osteomyelitis lesions can also be detected by ultrasonography. Tom turkeys are more affected than hens and affected birds have decreased cell‐mediated immunity.
E. coli are frequently isolated from the lesions, but other bacteria may also cause TOC lesions, especially Staphylococcus aureus, or S. hyicus. Culturing bones and livers from affected and unaffected birds in 2 turkey flocks resulted in recovery of pleomorphic, Gram‐variable bacteria consistent with L‐forms (cell‐wall‐deficient forms). Positive cultures were obtained more frequently from affected birds and bones than from unaffected birds or livers. The significance of these organisms in the disease is unknown, but the high number of isolates suggests these bacterial forms may be more common in turkeys than has been generally realized. Exposure of turkeys to low levels of E. coli via air sac inoculation increases the occurrence of TOC. The current hypothesis is that TOC is related less to the virulence of the infecting bacteria than it is to an inappropriate response of a subpopulation of male turkeys to stress, which increases their susceptibility to opportunistic bacterial infections. The greater susceptibility of turkeys selected for rapid growth and higher body weights to experimental TOC further supports the concept of genetic susceptibility that is most likely mediated by how the birds respond to stress. The protective effect of vitamin D3 also suggests a possible genetic basis for TOC susceptibility related to vitamin D receptors and their function. However, when vitamin D metabolites were administered, TOC was reduced as with vitamin D treatment, but there were toxic effects in dexamethasone‐treated turkeys challenged with E. coli (McMullin 2020).
Coligranuloma (Hjarre’s disease). Coligranuloma of chickens and turkeys is characterized by multiple granulomas in liver, ceca, duodenum, and mesentery, but not spleen (Figure 11). The disease also has been described in quail. Coligranuloma is an uncommon form of systemic colibacillosis that usually occurs sporadically in individual birds but can cause mortality as high as 75% when a flock is affected. Serosal lesions resemble leukosis tumors. Early in the disease there is confluent coagulation necrosis involving as much as half the liver. Only scattered heterophils are seen, and at the edge of the necrotic areas there are a few giant cells. Subsequently, typical heterophilic granulomas are present in the affected tissues. Pyogranulomatous typhlitis and hepatitis, which may be related to coligranuloma, have been described in turkeys with cecal cores and ruptured ceca. Recent studies attempting to replicate the disease using E. coli have failed to fulfil Koch’s postulates (McMullin 2020).
6.3. Morbidity and mortality
Both morbidity and mortality are highly variable depending on the type of disease produced by E. coli. It is probable that most, if not all, commercial flocks experience some degree of morbidity, mortality, or condemnation caused by E. coli infections. In flocks with highly virulent colisepticemia, it is occasionally possible to watch a bird sicken and die within a few hours. A flock that appears clinically normal when examined during the day but has an excess number of dead birds the following morning, is a common finding in mildly affected flocks. This pattern is typical for egg layer and breeder flocks experiencing coliform salpingitis/peritonitis (McMullin 2020).
7. Diagnosis
7.1. Differential diagnosis
Acute septicemic diseases may result from pasteurellae (Pasteurella, Ornithobacterium, Riemerella), salmonellae, streptococci, and other organisms. Chlamydophila, pasteurellae, or streptococci (Streptococcus, Enterococcus) can cause pericarditis or peritonitis, and other bacteria, mycoplasmas, and Chlamydophila can cause airsacculitis. Many organisms including viruses, mycoplasmas, and other bacteria can cause synovial lesions similar to those resulting from E. coli infection. A variety of organisms including Aerobacter spp., Klebsiella spp., Proteus spp., salmonellae, Bacillus spp., staphylococci, enterococci, or clostridia are frequently isolated (often as mixed cultures) from yolk sacs of embryos and chicks. Liver granulomas have many causes, including anaerobic bacteria belonging to the genera Eubacterium and Bacteroides (McMullin 2020).
7.2 Laboratory diagnosis
Diagnosis is based on isolation and identification of E. coli from lesions typical of colibacillosis. Care must be taken to avoid fecal contamination of samples. Isolation of the organism from visceral organs of birds undergoing decomposition must be interpreted cautiously as E. coli rapidly spreads from the intestinal tract into surrounding tissues of dead birds. Bone marrow cultures are easy to obtain and are generally free of contaminating bacteria. The brain is another site to culture, but it is less easily accessed compared with bone marrow (McMullin 2020).
Material should be streaked on EMB, MacConkey, or tergitol‐7 agar, as well as noninhibitory media. A presumptive diagnosis of E. coli infection can be made if most of the colonies are characteristically dark with a metallic sheen on EMB agar, bright pink, with a precipitate surrounding colonies on MacConkey agar, or yellow on tergitol‐7 agar. Strains of E. coli can be slow or nonlactose fermenters and appear as nonlactose‐fermenting colonies. Definitive identification of E. coli is based on the organism’s characteristics. A flow chart for the isolation and identification of E. coli has been published. A number of manual and automated systems are available for identification of bacteria, including E. coli (McMullin 2020).
Antigenic identification, determination of virulence factors, or fingerprinting of the isolate might be helpful, particularly when done as part of an epidemiologic investigation. The correlation between virulence and complement resistance suggests this may be a good method for screening isolates for possible disease association. A relatively simple rapid turbidimetric assay has been described. Also, analysis of the results of an extensive virulence genotyping assay identified 5 genes that typified a large majority of APEC. From these data, a pentaplex PCR “quick test” was designed to distinguish APEC from commensal E. coli isolated from the feces of healthy poultry without having to resort to in vivo virulence models. Also, a recent and extensive study identified 4 patterns of virulence genes for identifying APEC that could be exploited diagnostically. Despite the promise of these in vitro predictors of APEC identity, it may be unrealistic to expect them to identify all E. coli capable of causing avian colibacillosis. Most APEC are well equipped for a pathogenic lifestyle and are easily identified by their constellation of virulence genes; however, a few strains are not. Such “minority” isolates are likely opportunistic, causing disease in immunocompromised hosts and lacking the traits that gene‐based assays target.
Serology has not been used as a diagnostic method. However, survival after challenge correlated better with antibody titers detected by an enzyme‐linked immunosorbent assay (ELISA) than by the standard indirect hemagglutination procedure. Procedures to detect acute phase proteins or shifts in the heterophil/lymphocyte ratio can serve as nonspecific markers of the inflammation and stress that accompany colibacillosis.
8. Treatment
Antibiotics such as tetracyclines, sulfas, ampicillin, and streptomycin have been successfully used to control some E. coli. Early treatment is advised, with a follow-up considering antibiotic sensibility testing using the specific isolate. As the organism gets encapsulated or sequestered into caseous exudate, the antibiotic treatment will be less effective; thus, chronic stages of infection have a lower chance of being successfully treated.
No. | Product name | Dosage | Image |
1 | Prevention: 50g/ 50 liters of water or 50g/ 25kg of feed. Treatment: 50g/ 15 liters of water or 50g/ 5-10kg of feed.
| ||
2 | 3g/ 5kg body weight/day
| ||
4 | 1ml/5kg bw or 2ml/L of drinking water | ||
5 | 1 ml/2-4 liter of drinking water for 3-5 days. | ||
6 | 1ml/ liter of water. |
9.1 Control and prevention
Management practices designed to minimize the exposure level of these types of organisms in the bird’s environment are necessary in any preventive program. Avoiding overcrowding and providing proper ventilation along with good biosecurity measures, and appropriate sanitation and hygiene standards are highly effective in the control of colibacillosis in birds, and thereby reducing its zoonotic incidences. Prevention should be based on a good program of hygiene and sanitation meticulously from the nest to the chick box e.g. clean nests, frequent collection, sanitation of eggs, exclusion of severely soiled eggs, separate incubation of floor eggs etc. Follow good hatchery management practices. Immunosuppressive agents like infectious bursal disease (IBD), chicken infectious anaemia and aflatoxins should be checked, as they can flare up and increase the incidences of E. coli . Prevention of egg contamination by fumigating them within two hours after lay, and by removing cracked eggs or eggs soiled with faecal material is important step. Ultrasonic inactivation of E. coli followed by irradiation is one of the most efficient methods for preparation of effective vaccine against colibacillosis. BT-7, a piliated strain is effective as a live vaccine obtained from naturally occurring, non-pathogenic strain, used in chicken older than two weeks of age. Vitamins A, C, D, E along with selenium, β-carotene and iron can be given to improve the bird’s immune status. Contamination with APEC from the environment must be controlled by reduction and control of intestinal infection, best achieved using competitive exclusion (CE), i.e., inoculating day-old chicks with normal bacterial flora (probiotics) of healthy adult chickens. Birds also need to be protected against other pathogens that promote infections with APEC. For this appropriate disease prevention and control programs need to be followed like regular vaccinations for important poultry pathogens. The housing climate must be kept optimal for bird density, humidity, ventilation, dust and ammonia. The great diversity among APEC strains limits the possibilities of vaccination, and vaccines are not used on a large scale (Kuldeep, Sandip et al. 2013).
9.2 Vaccination
Before considering any E. coli vaccination, it is important to ensure that a timely and appropriate vaccination program is in place for likely primary agents, such as NDV, IBD, or hemorrhagic enteritis virus. A variety of E. coli vaccines and vaccination methods have been developed including passive and active immunization, use of inactivated and live products, recombinant and subunit vaccines, and immunization against specific virulence factors. No vaccination procedure has proved to be highly efficacious for multiple serotypes in the field at the present time. This is why broilers are rarely vaccinated. It is best to prevent the primary issue. However, in breeder hens, a killed autogenous E. coli vaccine is effective. Live vaccines against E. coli are popular amongst egg layer producers and have contributed to reducing E. coli peritonitis in layers.
Types of vaccines
Inactivated vaccines. Effective inactivated vaccines against various serotypes including O2:K1 and O78:K80 have been produced (Deb and Harry 1976, Deb and Harry 1978, Arp 1982). They provide protection against homologous serogroups, but no cross‐protection against heterologous serogroups. An inactivated O78 vaccine protected ducks (Sandhu and Layton 1985). Both homologous and heterologous protection were provided by a vaccine prepared by ultrasonic inactivation of the organism followed by irradiation (Melamed, Leitner et al. 1991). A vaccine containing bacterial membrane vesicles was effective in protecting turkey poults against challenge with pathogenic E. coli by stimulating antibody production, bacterial‐lysis activity of complement, T cell proliferation, and cytotoxic T cell activity (Chaffer, Schwartsburd et al. 1997). Use of a liposomal inactivated vaccine given by either eye drop or coarse spray stimulated humoral and mucosal antibodies. The number of bacteria in the blood was decreased and clinical signs were less severe in vaccinated birds following APEC challenge. Reported that an outer membrane protein vaccine offered a superior protection rate compared with other types of autogenous vaccines. Inactivated E. coli autogenous vaccines (aqueous and water‐in‐oil types) against E. coli peritonitis syndrome (EPS) in layers administered at 14 and 18 weeks induced an almost complete protection against homologous challenge. In laying hens, EPS is likely caused by non‐opportunistic virulent E. coli strains, making vaccination a good control option. The protective effect observed may be explained by the fact that vaccine‐induced systemic antibodies likely impede the access of E. coli to the bloodstream and/or favor its rapid clearance (Landman and Van Eck 2017).
Live vaccines. A live vaccine prepared from a naturally occurring, nonpathogenic, piliated E. coli strain (BT‐7) was efficacious when used in chickens older than 14 days of age. Protection against both homologous and heterologous strains was demonstrated (Frommer, Freidlin et al. 1994). E. coli J5, a mutant strain that has incomplete endotoxin in the cell wall exposing Gram‐negative core antigen, was both safe and effective for protecting chicks. Antibody titers to Gram‐negative core antigens that develop in commercial chickens peak during the pullet period (Ruble, Wakenell et al. 2002). In Japan, the productivity of field laying hens improved when ∆crp E. coli live vaccine was used (Uotani, Kitahara et al. 2017).
Recombinant and mutant vaccines. A carAB mutation of a virulent O2 serotype caused defective utilization of arginine and pyrimidines, increasing the requirements by the mutant. Because low levels of these substances are generally available in vivo, the organism was unable to sustain itself, which resulted in a self‐limiting infection. The mutant strain was found to be stable, immunogenic, and attenuated. Turkeys orally vaccinated with the mutant were protected against colibacillosis in a hemorrhagic enteritis virus‐parent wild‐type strain challenge model (Kwaga, Allan et al. 1994). Mutant O2 and O78 APEC with deletions of the genes cya or crp, which are involved in energy production, were used as a spray vaccine to immunize broiler chickens. The mutants were safe and immunogenic but provided only limited protection against airsacculitis following challenge (Peighambari, Hunter et al. 2002). Similarly, strain O78 mutants with deletions of galE, purA, and aroA genes were found to be safe and immunogenic, but provided only moderate protection against homologous challenge with no protection against heterologous challenge (Kariyawasam, Wilkie et al. 2004). In contrast to high mortality caused by the parent and wild‐type strains, attenuated streptomycin‐dependent (str‐dependent) mutants derived from a virulent APEC did not cause mortality in challenged birds. No protection against cellulitis or systemic lesions resulted when birds were vaccinated with high numbers of the mutant strains by aerosol and oral routes. However, systemic lesions were significantly reduced when birds were given three vaccinations on days 1 (aerosol), 14 (oral), and 28 (oral).
A recombinant vaccine using S. typhimurium was constructed to produce homologous group B determinants and E. coli O78 antigen. Vaccinated birds seroconverted and were protected against subsequent challenge with a pathogenic E. coli O78 strain (Roland, Curtiss III et al. 1999). A similar vaccine constructed to express E. coli type 1 fimbrial antigen in addition to O78 LPS provided protection against homologous challenge. The O78 LPS was responsible for most of the efficacy of the vaccine although presence of the fimbrial antigen did decrease the severity of air sac lesions. The fimbrial antigen did not provide cross-protection following challenge with O1 and O2 APEC serogroups (Roland, Karaca et al. 2004).
A modified‐live vaccine containing an aroA gene deleted E. coli, type O78, is licensed in the United States for use in chickens and turkeys by administration via drinking water or coarse spray (Poulvac E. coli, Pfizer). The vaccine significantly reduces mortality and lesions associated with E. coli, and provides cross‐protection for airsacculitis caused by E. coli serotypes O1, O2, and O18. A small study performed in Thailand did not find differences in mortality or morbidity between vaccinated and unvaccinated chickens, but lesions were significantly reduced in the vaccinated birds (Rawiwet and Chansiripornchai 2009). A recombinant multi-antigen vaccine has recently been tested in vitro and under experimental conditions, and appears to offer broad protection potential against avian pathogenic E. coli.
Molecular vaccines. Immunization of chickens with Iss, a surface protein common to APEC, but not commensal E. coli, is important in complement resistance, suggesting the potential to achieve cross‐protection among different serotypes (Lynne, Kariyawasam et al. 2012).
Chickens immunized with Iss produced humoral and mucosal antibody responses and provided good protection against colibacillosis following challenge with O1, O2, and O78 APEC serotypes. Challenged birds had significantly lower lesion scores compared with unvaccinated, challenged controls (Lynne, Kariyawasam et al. 2012). Multivalent vaccines made from pili containing low levels (180μg) of protein per dose reduced the severity of challenge infection (Gyimah, Panigrahy et al. 1986). Absorbed sera indicate pili of serotypes O1, O2, and O78 are antigenically different (Suwanichkul, Panigrahy et al. 1987). Fimbrial vaccines containing FimH, the adhesin of F1A (type 1) fimbriae, or PapGII, the highly conserved portion of P fimbrial adhesion, were immunogenic but did not provide protection against APEC challenge. The results of PapGII immunization differ from the finding that passive immunization with PapG yolk‐derived antibodies was protective (see Passive Immunization).
Passive immunization. Passive immunization results in increased resistance to aerosol challenge and clearance of bacteria from blood (Myers and Arp 1987). Use of inactivated vaccines in breeders provided passive protection against homologous challenge in progeny, which was complete for 2 weeks and partial for several additional weeks post-hatch (Heller, Leitner et al. 1990).
Antiserum prepared in rabbits against iron‐regulated outer membrane proteins of E. coli protected turkeys against mortality following challenge. Frequency of bacteremia at 96 hours after challenge, recovery of E. coli from air sacs, and severity of gross lesions were significantly reduced in immunized birds compared with control birds given normal rabbit serum or saline solution (Bolin and Jensen 1987).
Antibodies extracted from the yolk of eggs laid by immunized hens provided homologous protection against an O78 APEC. Partial protection against heterologous challenge with O1 and O2 serotypes was provided by immunizing hens with P pilus adhesin (PapG) or the aerobactin outer membrane receptor IutA. Immunizing with PapG provided the best overall protection. Breeder hens immunized by this method may provide immunity to their progeny (Kariyawasam, Wilkie et al. 2004).
Immuno-potentiation. A problem with recombinant vaccines is low immunogenicity, which could potentially be solved by using effective immunopotentiators. Inoculation of chickens by intramuscular or subcutaneous routes and chicks by intramuscular or in ovo routes with cytosine‐phosphodiester‐guanine (CpG) oligodeoxy-nucleotides improved livability and reduced cellulitis lesion size following challenge with APEC (Gomis, Babiuk et al. 2003, Gomis, Babiuk et al. 2004). Use of CpG as an adjuvant in an inactivated E. coli vaccine improved the efficacy of the vaccine (Gomis, Babiuk et al. 2007). CpG motifs are present in high numbers in bacterial DNA and enhance innate immune responses (Babiuk, Gomis et al. 2003, Gomis, Babiuk et al. 2004).
Modification of E. coli heat‐labile enterotoxin (LT) resulted in a nontoxic protein (nLT) that stimulated antibody production in chickens following either oral or parenteral co‐administration of an antigen to chickens (Vasserman and Pitcovski 2006).
Mixtures of antimicrobial/host defense peptides and gamma‐amino‐butyric acid promoted production of proinflammatory cytokines following subcutaneous injection. Chickens challenged with a virulent O2 APEC 24 hours after receiving the mixture had lower mortality and bacteremia, but there was no effect on cellulitis lesions. Intramuscular administration was less effective (Allan, Buchanan et al. 2012).
10. References
Allan, B., et al. (2012). "Innate immune cocktail partially protects broilers against cellulitis and septicemia." Avian diseases 56(4): 659-669.
Antao, E.-M., et al. (2008). "The chicken as a natural model for extraintestinal infections caused by avian pathogenic Escherichia coli (APEC)." Microbial pathogenesis 45(5-6): 361-369.
Babiuk, L., et al. (2003). "Molecular approaches to disease control." Poultry science 82(6): 870-875.
Barbieri, N. L., et al. (2012). "Characterization of extraintestinal Escherichia coli isolated from a peacock (Pavo cristatus) with colisepticemia." Avian diseases 56(2): 436-440.
Bhunia, A. K. (2018). Foodborne microbial pathogens: mechanisms and pathogenesis, Springer.
Bisgaard, M. (1995). "Salpingitis in web‐footed birds: prevalence, aetiology and significance." Avian pathology 24(3): 443-452.
Burns, K. E., et al. (2003). "Cellulitis in Japanese quail (Coturnix coturnix japonica)." Avian diseases 47(1): 211-214.
Chaffer, M., et al. (1997). "Vaccination of turkey poults against pathogenic Escherichia coli." Avian Pathology 26(2): 377-390.
Chamanza, R., et al. (1999). "Acute phase proteins in the domestic fowl." World's Poultry Science Journal 55(1): 61-71.
Crespo, R., et al. (2001). "Salpingitis in Pekin ducks associated with concurrent infection with Tetratrichomonas sp. and Escherichia coli." Journal of veterinary diagnostic investigation 13(3): 240-245.
De Boever, S., et al. (2009). "Characterization of an intravenous lipopolysaccharide inflammation model in broiler chickens." Avian Pathology 38(5): 403-411.
DeRosa, M., et al. (1992). "Acute airsacculitis in untreated and cyclophosphamide-pretreated broiler chickens inoculated with Escherichia coli or Escherichia coli cell-free culture filtrate." Veterinary pathology 29(1): 68-78.
Dho-Moulin, M. and J. M. Fairbrother (1999). "Avian pathogenic Escherichia coli (APEC)." Veterinary research 30(2-3): 299-316.
Díaz-Sánchez, S., et al. (2012). "Occurrence of avian pathogenic Escherichia coli and antimicrobial-resistant E. coli in red-legged partridges (Alectoris rufa): sanitary concerns of farming." Avian pathology 41(4): 337-344.
Dziva, F. and M. P. Stevens (2008). "Colibacillosis in poultry: unravelling the molecular basis of virulence of avian pathogenic Escherichia coli in their natural hosts." Avian Pathology 37(4): 355-366.
Edelman, S., et al. (2003). "In vitro adhesion of an avian pathogenic Escherichia coli O78 strain to surfaces of the chicken intestinal tract and to ileal mucus." Veterinary microbiology 91(1): 41-56.
Elfadil, A., et al. (1996). "Description of cellulitis lesions and associations between cellulitis and other categories of condemnation." Avian diseases: 690-698.
Frommer, A., et al. (1994). "Experimental vaccination of young chickens with a live, non‐pathogenic strain of Escherichia coli." Avian Pathology 23(3): 425-433.
Gibbs, P. S., et al. (2004). "Comparison of several challenge models for studies in avian colibacillosis." Avian diseases 48(4): 751-758.
Gomis, S., et al. (2004). "Protection of neonatal chicks against a lethal challenge of Escherichia coli using DNA containing cytosine-phosphodiester-guanine motifs." Avian diseases 48(4): 813-822.
Gomis, S., et al. (2007). "Protection of chickens against a lethal challenge of Escherichia coli by a vaccine containing CpG oligodeoxynucleotides as an adjuvant." Avian diseases 51(1): 78-83.
Gomis, S., et al. (2003). "Protection of chickens against Escherichia coli infections by DNA containing CpG motifs." Infection and immunity 71(2): 857-863.
Guabiraba, R. and C. Schouler (2015). "Avian colibacillosis: still many black holes." FEMS microbiology letters 362(15): fnv118.
Harmon, B. G. (1998). "Avian heterophils in inflammation and disease resistance." Poultry science 77(7): 972-977.
Heller, E., et al. (1990). "Passive immunisation of chicks against Escherichia coli." Avian Pathology 19(2): 345-354.
Hines, M. E., et al. (1995). "Combined adenovirus and rotavirus enteritis with Escherichia coli septicemia in an emu chick (Dromaius novaehollandiae)." Avian diseases: 646-651.
Hoque, M., et al. (2012). "Causes of morbidity and mortality of wild aquatic birds at Billabong Sanctuary, Townsville, North Queensland, Australia." Avian diseases 56(1): 249-256.
Horn, F., et al. (2012). "Infections with avian pathogenic and fecal Escherichia coli strains display similar lung histopathology and macrophage apoptosis." PloS one 7(7): e41031.
Johnson, L. C., et al. (2001). "The influence of Escherichia coli strains from different sources and the age of broiler chickens on the development of cellulitis." Avian Pathology 30(5): 475-478.
Jordan, F., et al. (2005). "Observations on salpingitis, peritonitis and salpingoperitonitis in a layer breeder flock." Veterinary record 157(19): 573-577.
Kariyawasam, S., et al. (2004). "Construction, characterization, and evaluation of the vaccine potential of three genetically defined mutants of avian pathogenic Escherichia coli." Avian diseases 48(2): 287-299.
Kariyawasam, S., et al. (2004). "Resistance of broiler chickens to Escherichia coli respiratory tract infection induced by passively transferred egg-yolk antibodies." Veterinary microbiology 98(3-4): 273-284.
Kathayat, D., et al. (2021). "Avian Pathogenic Escherichia coli (APEC): An Overview of Virulence and Pathogenesis Factors, Zoonotic Potential, and Control Strategies." Pathogens 10(4): 467.
Knöbl, T., et al. (2001). "Virulence properties of Escherichia coli isolated from ostriches with respiratory disease." Veterinary microbiology 83(1): 71-80.
Kuldeep, D., et al. (2013). "Escherichia coli, an economically important avian pathogen, its disease manifestations, diagnosis and control, and public health significance: a review." Research Opinions in Animal and Veterinary Sciences 3(6): 179-194.
Kwaga, J., et al. (1994). "A carAB mutant of avian pathogenic Escherichia coli serogroup O2 is attenuated and effective as a live oral vaccine against colibacillosis in turkeys." Infection and immunity 62(9): 3766-3772.
La Ragione, R., et al. (2000). "The role of fimbriae and flagella in the colonization, invasion and persistence of Escherichia coli O78 [ratio] K80 in the day-old-chick model." Epidemiology & Infection 124(3): 351-363.
Landman, W. and J. Van Eck (2017). "The efficacy of inactivated Escherichia coli autogenous vaccines against the E. coli peritonitis syndrome in layers." Avian Pathology 46(6): 658-665.
Litjens, J., et al. (1989). "A case of swollen head syndrome in a flock of guinea fowl." Tijdschrift voor diergeneeskunde 114(13): 719-720.
Lynne, A. M., et al. (2012). "Recombinant Iss as a potential vaccine for avian colibacillosis." Avian diseases 56(1): 192-199.
Matter, L. B., et al. (2011). "Avian pathogenic Escherichia coli MT78 invades chicken fibroblasts." Veterinary microbiology 148(1): 51-59.
McMullin, P. F. (2020). Diseases of poultry 14th edition: David E. Swayne, Martine Boulianne, Catherine M. Logue, Larry R. McDougald, Venugopal Nair, David L. Suarez, Sjaak de Wit, Tom Grimes, Deirdre Johnson, Michelle Kromm, Teguh Yodiantara Prajitno, Ian Rubinoff & Guillermo Zavala (Eds.), Hoboken, NJ, John Wiley & Sons, 2020, 1451 pp.,£ 190 (hardcover)/£ 171.99 (e-book), ISBN 9781119371168, Taylor & Francis.
Melamed, D., et al. (1991). "A vaccine against avian colibacillosis based on ultrasonic inactivation of Escherichia coli." Avian diseases: 17-22.
Mellata, M. (2013). "Human and avian extraintestinal pathogenic Escherichia coli: infections, zoonotic risks, and antibiotic resistance trends." Foodborne pathogens and disease 10(11): 916-932.
Miller, D. L., et al. (2004). "An Escherichia coli epizootic in captive mallards (Anas platyrhynchos)." International Journal of Poultry Science 3(3): 206-210.
Mireles, A., et al. (2005). "An acute inflammatory response alters bone homeostasis, body composition, and the humoral immune response of broiler chickens." Poultry Science 84(4): 553-560.
Montgomery, R. D., et al. (1999). "Consequences to chicks hatched from Escherichia coli-inoculated embryos." Avian diseases: 553-563.
Montgomery, R. D., et al. (2005). "The embryo lethality of Escherichia coli isolates and its relationship to various in vitro attributes." Avian diseases 49(1): 63-69.
Nakamura, K., et al. (1998). "Serum levels of interleukin-6, alpha1-acid glycoprotein, and corticosterone in two-week-old chickens inoculated with Escherichia coli lipopolysaccharide." Poultry Science 77(6): 908-911.
Peighambari, S., et al. (2002). "Safety, immunogenicity, and efficacy of two Escherichia coli cya crp mutants as vaccines for broilers." Avian diseases 46(2): 287-297.
Pourbakhsh, S. A., et al. (1997). "Dynamics of Escherichia coli infection in experimentally inoculated chickens." Avian diseases: 221-233.
Pourbakhsh, S. A., et al. (1997). "Localization of thein vivoexpression of P and F1 fimbriae in chickens experimentally inoculated with pathogenicEscherichia coli." Microbial pathogenesis 22(6): 331-341.
Ramírez, R. M., et al. (2009). "Adherence and invasion of avian pathogenic Escherichia coli to avian tracheal epithelial cells." World Journal of Microbiology and Biotechnology 25(6): 1019-1023.
Ramírez, R. M., et al. (2009). "Avian pathogenic Escherichia coli bind fibronectin and laminin." Veterinary research communications 33(4): 379-386.
Raue, R., et al. (2005). "A disease complex associated with pigeon circovirus infection, young pigeon disease syndrome." Avian Pathology 34(5): 418-425.
Rawiwet, V. and N. Chansiripornchai (2009). "The efficacy of Escherichia coli aroA-live vaccine in broilers against avian E. coli serotype O78 infection." The Thai Journal of Veterinary Medicine 39(4): 337-342.
Roland, K., et al. (1999). "Construction and evaluation of a Δcya Δcrp Salmonella typhimurium strain expressing avian pathogenic Escherichia coli O78 LPS as a vaccine to prevent airsacculitis in chickens." Avian diseases: 429-441.
Roland, K., et al. (2004). "Expression of Escherichia coli antigens in Salmonella typhimurium as a vaccine to prevent airsacculitis in chickens." Avian diseases 48(3): 595-605.
Ruble, R. P., et al. (2002). "Seroprevalence of antibodies specific for gram-negative core antigens in chickens on the basis of an Escherichia coli J5 enzyme-linked immunosorbent assay." Avian diseases 46(2): 453-460.
Sugiarto, H. and P.-L. Yu (2006). "Identification of three novel ostricacins: an update on the phylogenetic perspective of β-defensins." International journal of antimicrobial agents 27(3): 229-235.
Tonu, N., et al. (2011). "Pathologicalstudy on colibacillosis in chickens and detection of Escherichia coli by PCR." Bangladesh Journal of Veterinary Medicine 9(1): 17-25.
Uotani, Y., et al. (2017). "Efficacy of an avian colibacillosis live vaccine for layer breeder in Japan." Journal of Veterinary Medical Science: 17-0189.
Vandekerchove, D., et al. (2005). "Virulence-associated traits in avian Escherichia coli: comparison between isolates from colibacillosis-affected and clinically healthy layer flocks." Veterinary microbiology 108(1-2): 75-87.
Vasserman, Y. and J. Pitcovski (2006). "Genetic detoxification and adjuvant-activity retention of Escherichia coli enterotoxin LT." Avian Pathology 35(02): 134-140.
Wooley, R., et al. (2000). "Chicken embryo lethality assay for determining the virulence of avian Escherichia coli isolates." Avian diseases: 318-324.
Xie, H., et al. (2002). "Changes in serum ovotransferrin levels in chickens with experimentally induced inflammation and diseases." Avian Diseases 46(1): 122-131.
Zanella, A., et al. (2000). "Severe Escherichia coli O111 septicaemia and polyserositis in hens at the start of lay." Avian Pathology 29(4): 311-317.
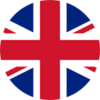