To My Quyen, Tran Duy Thanh,
Nguyen Khanh Thuan, Nguyen Phuc Khanh, Nguyen Thanh Lam*
1. Introduction
Salmonella infections in poultry flocks can cause acute and chronic clinical diseases but have received greater international attention in recent years because of their role in foodborne outbreaks of human illness. Contaminated poultry meat and eggs are among the most frequently implicated food vehicles of Salmonellae. As a consequence of both public health and flock health concerns, Salmonella infections cause economically significant losses for poultry producers in many nations and absorb large investments of government and private resources for testing and control efforts. Accordingly, the present edition of this chapter focuses on these food‐transmissible “paratyphoid” Salmonellae. Clinical signs and gross lesions associated with Salmonella are not completely distinctive from other bacterial infections, so diagnosis generally requires the isolation or identification of causative organisms. Effective strategies for controlling these zoonotic pathogens require the sustained implementation of comprehensive risk reduction practices throughout the production continuum (Gast and Porter Jr., 2020).
2. Aetiology
2.1 Bacterial characteristics
Salmonellae are straight, nonspore‐forming rods, measuring about 0.7–1.5×2.0–5.0μm. Salmonellae are Gram‐negative, but cells can readily be stained with common dyes such as methylene blue or carbolfuchsin. Paratyphoid Salmonella are usually peritrichously flagellated and motile, although naturally occurring nonmotile mutants are occasionally encountered. Both S. Pullorum and S. Gallinarum are characteristically nonmotile.
Salmonellae are facultatively anaerobic and can grow well under both aerobic and anaerobic conditions. The optimum temperature to support Salmonella multiplication is 37°C, but some growth is observed over a range from about 5°C to 45°C. Salmonellae can grow within a pH range of approximately 4.0–9.0, with an optimum around 7.0, although cellular components such as flagella and fimbria may not be expressed under extreme pH conditions. The nutritional requirements of Salmonellae are relatively simple, and most culture media that supply sources of carbon and nitrogen can support their growth. The viability of Salmonella cultures can be maintained for many years in simple media, such as peptone agar or nutrient agar, which have been stab‐inoculated, sealed, and held at room temperature.
Typical paratyphoid Salmonellae ferment glucose (to produce both acid and gas), dulcitol, mannitol, maltose, and mucate, but do not ferment lactose, sucrose, malonate, or salicin. However, some S. Arizonae strains are able to ferment lactose slowly but may not utilize dulcitol. Most Salmonella strains can produce hydrogen sulfide on many types of media, decarboxylate ornithine and lysine, utilize citrate as a sole source of carbon, and reduce nitrates to nitrites. Paratyphoid Salmonellae do not hydrolyze urea or gelatin and do not produce indole. Most paratyphoid Salmonellae can be readily distinguished from the avian host‐adapted biovars, S. Pullorum and S. Gallinarum, on the basis of the inability of S. Pullorum strains to ferment mucate or dulcitol and the inability of S. Gallinarum strains to decarboxylate ornithine or produce gas from glucose fermentation. In addition, para- typhoid Salmonellae are usually motile but S. Pullorum and S. Gallinarum are typically nonmotile (Gast and Porter Jr., 2020).
2.2 Classification
The genus Salmonella is a member of the family Enterobacteriaceae and consists of 2 genetically distinct species, one of which (S. enterica) includes 6 subspecies determined by patterns of biochemical reactions. Only 1 subspecies (S. enterica subspecies enterica) is associated with disease in warm‐blooded animals and includes more than 2,500 motile and nonhost‐adapted serovars such as S. enterica subspecies enterica serovar Enteritidis and S. enterica subspecies enterica serovar Typhimurium. The traditional serovar designations (S. Enteritidis, S. Typhimurium) are routinely employed for concise diagnostic classification and epidemiologic analysis. Although the causative agents of pullorum disease and fowl typhoid have been taxonomically assigned to a single serovar, S. Pullorum‐Gallinarum, these 2 organisms are genetically and biochemically distinguishable and the 2 biovars are accordingly more commonly designated separately as S. Pullorum and S. Gallinarum (Gast and Porter Jr., 2020).
The genus Salmonella of the family Enterobacteriaceae can roughly be classified into three categories or groups (Hafez, 2001):
Group 1, highly host adapted and invasive serovars. This group includes species restricted and invasive Salmonella such as S. Pullorum, S. Gallinarum in poultry and S. Typhi in humans.
Group 2, non-host adapted and invasive serovars. This group consists of approximately 10-20 serovars that are able to cause an invasive infection in poultry and may be capable of infecting humans. Currently, the most important serovars are S. Typhimurium, S. Hadar, S. Arizonae and S. Enteritidis.
Group 3, non-host adapted and non-invasive serovars. Most serovars of the genus Salmonella belong to this group and are harmless for animals and humans.
2.3 Resistance
Environmental persistence by paratyphoid Salmonellae creates continuous opportunities for horizontal transmission of infection within and between flocks. S. Enteritidis has been observed to survive in litter and feed for 26 months after removal of an infected flock. S. Senftenberg persisted for more than 2 years in a poultry house despite depopulation, cleaning, disinfection, drying, and numerous intervening negative environmental tests. High moisture levels are particularly important risk factors for Salmonella persistence. The numbers of viable Salmonella in poultry litter are directly related to water activity and accordingly tend to increase in regions of houses with reduced airflow. Reducing water activity or manipulating pH to antibacterial extremes have reduced Salmonella populations in poultry litter (Payne et al., 2007). Strains of S. Pullorum and S. Gallinarum are also capable of extended survival under favorable environmental conditions, although they are generally less resistant to heat and chemicals than most paratyphoid Salmonellae.
3. Pathogenicity
3.1 Toxin
Two general categories of toxins play roles in the pathogenicity of Salmonellae. Endotoxin is associated with the lipid A portion of Salmonella cell wall lipopolysaccharide (LPS). If released into the bloodstream of an infected animal upon lysis of bacterial cells, endotoxin can produce fever. Intravenously administered S. Enteritidis endotoxin caused liver and spleen lesions in 2‐week‐old chickens. LPS also contributes to the resistance of the bacterial cell wall to attack and digestion by host phagocytes. Loss of the ability to synthesize complete LPS impairs the ability of S. Typhimurium to colonize the ceca and invade the spleen in broiler chicks. Several proteinaceous toxins have also been identified in Salmonella. Enterotoxin activity induces a secretory response by epithelial cells that results in fluid accumulation in the intestinal lumen. The heat‐labile cytotoxin causes structural damage to intestinal epithelial cells, perhaps by inhibiting protein synthesis (Gast and Porter Jr., 2020).
3.2 Adherence and invasion
Adherence to intestinal epithelial cells is the pivotal first step in the sequence of events by which Salmonellae cause disease. Inefficient colonization of the intestinal tracts of chicks by Salmonella strains correlates with severely attenuated virulence. Variations between serovars in their persistence in chicken ceca have been attributed to differential regulation of core motility and adherence genes. Several characterized Salmonella virulence genes are upregulated in the high‐osmolarity intestinal environment. Both flagella and fimbria have been identified as mediators of intestinal attachment, although neither are absolutely essential. Mutants of S. Enteritidis lacking flagella exhibited reduced adherence to cultured avian intestinal cells and competed poorly with wild‐type strains to colonize the ceca of chicks. Strains of S. Enteritidis lacking fimbria were isolated less often than fimbriated strains from the ceca of inoculated chicks. LPS may also play a role in gastrointestinal attachment by Salmonellae. Salmonella virulence also requires mucosal invasion following adherence. Adherence and invasion appear to be separately regulated activities. The expression of important virulence genes in Salmonella pathogenicity island 1 strongly influences invasion of internal tissues such as livers and spleens but has much less effect on initial cecal colonization. Mutations affecting intestinal colonization and lethality after oral inoculation of chicks did not have similar effects after intra-peritoneal administration. Flagella and some types of fimbria may play roles in S. Enteritidis invasion and dissemination to internal organs of chicks. Flagella‐deficient (but not fimbria‐deficient) mutants were less able to invade to the livers and spleens of chicks. Type 1 fimbria appear to mediate the colonization of tubular gland cells in the upper oviduct. Both S. Pullorum and S. Gallinarum have lost the functionality of flagella and other genes essential to efficient colonization of the gut, so their virulence is accordingly more highly dependent on survival and multiplication in internal tissues (Gast and Porter Jr., 2020).
4. Epidemiology
4.1 Incidence of Salmonella serovars
Numerous and varied estimates have been made of the incidence of Salmonellae in poultry and poultry house environments around the world. Recent surveys of the incidence of Salmonella infection among turkey flocks have ranged from 16% to 54%, with 20% of breeding flocks also reported as positive. Likewise, recent surveys of the incidence of Salmonella infection among broiler chicken flocks have ranged from 9% to 57%, with up to 47% of breeding flocks also identified as positive. Incidences of infection can differ substantially across national borders, even within the same geographic region, and national Salmonella isolation rates can sometimes change considerably from year to year. The actual prevalence of infection or contamination within Salmonella‐positive flocks can also vary widely. For example, within turkey flocks, Salmonellae were isolated from 13% of litter samples and 11% of cecal samples in 1 study and 79% of litter samples and 70% of fecal samples in another. Moreover, the incidence of infection or contamination among flocks does not necessarily correlate with either the prevalence of infection within flocks or the quantitative level of contamination within poultry facilities. Recent surveys for the incidence of Salmonella in egg‐type poultry in various nations have generated similarly diverse results, ranging from 12% to 65% of laying flocks and up to 26% of breeding flocks. The frequency of Salmonella‐positive samples within egg-laying flocks is often far less than the overall incidence among flocks (Gast and Porter Jr., 2020).
4.2 Distribution of Salmonella serovars
The distribution of serovars from poultry sources varies geographically and changes over time, although several serovars are consistently found at a high incidence. Among clinical and environmental isolates submitted in the United States in 2014, the most frequently identified serovars were S. Senftenberg, S. Kentucky, S. Mbandaka, S. Enteritidis, S. Typhimurium, and S. Infantis in chickens; and S. Senftenberg, S. Anatum, S. Hadar, S. Muenster, S. Agona, and S. Heidelberg in turkeys. The important epidemiological connection between poultry and human reservoirs of Salmonellae is sometimes evident in similar serovar distributions. For example, S. Enteritidis and S. Typhimurium, which have both been highly prevalent in poultry for many years, were the most frequently isolated serovars from humans in the United States in 2012. The implementation of new food safety regulations in Australia changed the relative incidences of Salmonella serovars, but not the linkage between the serovars found in poultry and those causing human illness (Sumner et al., 2004).
Reports of the frequencies at which paratyphoid Salmonellae are isolated from poultry sources around the world vary widely, although several serovars are of continuing international significance. The unique epidemiologic association of S. Enteritidis with transmission of disease to consumers via contaminated eggs has made the prevalence of this serovar a topic of particular interest. S. Enteritidis has been the most common serovar found in surveys of egg‐producing chickens in many nations and has been reported as the principal serovar present in eggs even when other serovars are predominant in associated laying flocks (Otomo et al., 2007). S. Enteritidis has also become a prominent isolate from broiler chickens in some countries. Several other serovars are highly prevalent in particular types of poultry or certain geographic regions. Serovar Typhimurium has been reportedly associated with the widest variety of commercial poultry species. An increased prevalence of S. Kentucky has been recently observed in both egg‐type and meat‐type poultry in the United States. S. Infantis has been common in both layers and broilers in Japan. S. Sofia has predominated for a number of years in Australian poultry. Other widely prevalent serovars in poultry include S. Heidelberg, S. Hadar, S. Mbandaka, and S. Anatum. The host‐adapted pathogens S. Pullorum and S. Gallinarum have rarely been reported in recent decades from economically developed regions such as the United States, Canada, Western Europe, and Australia, except in sporadic instances from backyard poultry flocks. However, in Asia and Latin America, these organisms (and the diseases they cause) remain highly prevalent in both subsistence and commercial flocks.
5. Susceptible hosts
5.1 Salmonella infections in chicks and poults
Salmonella infections often have far different consequences for newly hatched poultry than for older birds. In very susceptible young chicks and poults, Salmonella infection can sometimes lead to illness and death at high frequencies. For example, oral doses of 109 S. Typhimurium cells were lethal for 50% of broiler chicks at 1 day of age and 20% at 3 days, but for 0% at 7 days. Mortality associated with naturally occurring infections in poultry often reaches peak levels at 3–7 days of age. Older birds are much less susceptible to the lethal effects of Salmonellae and may experience intestinal colonization and even systemic dissemination without significant morbidity or mortality. Mortality associated with S. Pullorum infection is generally confined to the first 2–3 weeks of life, although birds that survive early infection may become inapparent carriers of the pathogen in splenic macrophages and the reproductive tract (Wigley et al., 2001). S. Arizonae infections are sometimes associated with high morbidity and mortality in young turkey poults. The development of resistance to Salmonellae in young birds is mostly attributable to the acquisition of protective microflora from their feed and environment. These organisms compete with Salmonellae for intestinal receptor sites or produce inhibitory factors. Significantly more orally administered S. Typhimurium cells adhered in the ceca of chicks at 2 days of age than at 3–7 days. Naturally exposed birds can be heavily infected on their first day of life, and the frequency of fecal shedding of Salmonellae in broiler flocks has been reported to peak at 14 days of age. Only 4% of cecal samples collected from naturally infected turkey poults were Salmonella‐positive at 1 day of age, but 55% were positive at 9 days (Wesley et al., 2011).
Salmonella infection has been experimentally established by oral, intracloacal, intratracheal, intraocular, navel, and aerosol administration to chicks. The usual outcomes of paratyphoid infections in chicks and poults involve 3 stages. Orally introduced Salmonellae first establish intestinal colonization, often resulting in persistent fecal shedding. Salmonella levels in the intestinal contents of colonized chicks may greatly exceed intracellular levels in the intestinal epithelium. Second, invasion beyond the gastrointestinal tract can lead to Salmonella multiplication in the macrophage–phagocyte system of the liver and spleen, and eventual dissemination to colonize a variety of internal tissue sites. Third, extensive bacteremia sometimes occurs, resulting in high mortality. The bacterial incidence at all individual stages of infection correlates strongly with the dose of orally administered Salmonellae. Infectious doses of Salmonellae may vary between different avian species. Unlike paratyphoid strains which typically cause rapid intestinal inflammation in the early stages of infection, S. Pullorum has been shown to preferentially target sites such as the bursa of Fabricius (Henderson et al., 1999).
Salmonella intestinal colonization, invasion to internal organs, and persistence in colonized tissues are all higher in newly hatched chicks than in older birds. Intestinal persistence was far greater following oral inoculation of chicks with S. Typhimurium or S. Enteritidis at 1 day of age than at 7 days. After oral inoculation of 1‐day‐old chicks, S. Enteritidis was shed in the feces of nearly half of these birds at 6 months of age. Salmonella infection has been reported to persist for as long as 64 weeks after the exposure of young chicks (Phillips et al., 1995). Administering 102 S. Enteritidis cells to 1‐day‐old chicks led to more persistent intestinal infection than administering 109 cells to 1‐week‐old birds. Some internal tissues reportedly remained Salmonella‐positive for as long as 1 year after inoculation of 1‐day‐old chicks (Skov et al., 2002).
5.2 Salmonella infections in older poultry
Morbidity or mortality are not consistently associated with paratyphoid Salmonella infections in older poultry, although fowl typhoid can sometimes cause significant disease losses in adult flocks. Experimental infections of adult chickens with large oral doses of most serovars may result in extensive intestinal colonization, bacteremia, and systemic dissemination to diverse internal organs, but they rarely cause any evident signs of clinical illness. During the first 2–4 weeks following experimental oral infection of adult chickens or turkeys, Salmonellae are typically isolated from intestinal tracts and voided feces at high frequencies. Although the incidence of gut colonization and fecal shedding steadily decline thereafter, some S. Enteritidis strains can persist in the intestines of chickens for several months after oral inoculation. Salmonella was isolated from 42% of commercial broilers at 6–8 weeks of age. The frequency of fecal shedding of Salmonellae by commercial layers peaked at 18 weeks of age and then declined steadily.
Gut colonization by Salmonellae is usually followed by invasion through the intestinal epithelium and dissemination to colonize internal tissues. In commercial broilers, 35% of sampled spleens, livers, and gall bladders were Salmonella‐positive at 6–8 weeks of age. Various serovars (including S. Typhimurium and S. Heidelberg) are known to be invasive for poultry, but the patterns and consequences of systemic invasion have been documented most extensively for S. Enteritidis. After experimental oral inoculation of laying hens, S. Enteritidis has been isolated from numerous internal tissues, including the liver, spleen, ovary, oviduct, heart blood, and peritoneum. Dissemination of S. Enteritidis to diverse internal organs, including the ovary and oviduct, has also been recorded following intravenous, intratracheal, conjunctival, intravaginal, or intracloacal inoculation, exposure to contaminated aerosols, or insemination with contaminated semen. The magnitude of internal organ colonization is directly related to the infecting dose. S. Enteritidis has also been isolated from a wide range of internal organs in naturally infected poultry.
The production of contaminated eggs is an aspect of Salmonella infections in mature chickens which has unique public health ramifications. Among egg‐transmitted human salmonellosis outbreaks, 74% have been caused by S. Enteritidis. Investigations of egg‐laying flocks implicated in human disease outbreaks have sometimes found S. Enteritidis isolates of the same phage types, plasmid profiles, or chromosomal DNA profiles found in affected people. Internal contamination of eggs with S. Enteritidis is a consequence of invasion to the ovary and oviduct. S. Enteritidis can colonize diverse regions of the reproductive tract of chickens, although the relationship between the colonization site and the frequency or location of egg contamination is uncertain. Both S. Heidelberg and S. Typhimurium have also been found inside eggs laid by experimentally infected hens. However, some strains of these serovars can colonize reproductive organs but are rarely deposited in eggs. The reported incidence of S. Enteritidis contamination of eggs from infected commercial flocks has usually been extremely low, even when laying house environments are contaminated, but transiently higher incidences may sometimes lead to human disease outbreaks. After oral inoculation of hens, S. Enteritidis can be deposited in both yolk and albumen, although usually at very low initial concentrations. Naturally contaminated eggs also typically contain very small numbers of S. Enteritidis cells, but these populations can expand to more dangerous levels if eggs are held at growth‐promoting temperatures (Gast and Porter Jr., 2020).
5.3 Predisposing factors
Several other infectious agents are known to influence the course of Salmonella infections. Prior infection with coccidia such as Eimeria tenella can increase Salmonella colonization of the intestinal tracts of chickens and E. tenella exposure may cause recrudescence of an earlier Salmonella infection. Coccidial infection decreases intestinal levels of Salmonella‐inhibiting volatile fatty acids. However, E. tenella infection also diminished the frequency at which subsequently administered S. Enteritidis invaded to the internal organs of chicks, possibly by thickening the intestinal lamina propria. Infections with immunosuppressive viruses or bacteria can also affect the outcome of Salmonella infections in poultry. Exposure to reticuloendotheliosis virus or bursal disease virus at 1 day of age increased mortality among chicks inoculated subsequently with Salmonellae. Suppression of cell‐mediated immunity by Corynebacterium parvum led to increased morbidity in chicks after Salmonella infection. Perhaps via protection against compromising infections, both coccidial and viral vaccines have been associated with reduced Salmonella detection in broiler flocks.
Environmental and management factors also influence the susceptibility of poultry to Salmonellae. Stressful conditions facilitate or exacerbate infections. Lowering the brooding temperature of chicks by 5–8°C increased mortality among newly hatched chicks inoculated with S. Worthington. Stress from environmental heat or cold, water deprivation, and the onset of egg laying have all been associated with higher frequencies or duration of Salmonella isolation from experimentally infected chickens. The incidence of Salmonella in broiler chickens sometimes increases after they are transported for processing. Both preslaughter feed withdrawal and exposure to heat alter intestinal morphology and microbial communities and increase Salmonella attachment to intestinal cells. Inducing molting by feed restriction has been identified as a highly significant risk factor for S. Enteritidis infection in laying flocks. Feed deprivation lowers crop levels of protective lactobacilli and volatile fatty acids while increasing pH. In experimental infection studies, feed restriction of hens increased intestinal colonization and fecal shedding, intestinal lesions, invasion to livers and spleens, recurrence of prior infections, and horizontal or airborne transmission of S. Enteritidis infection. Molting can also be induced via alternatives such as fiber‐based diets without affecting Salmonella susceptibility.
A diverse assortment of poultry facility characteristics and management practices, including larger flock size, greater flock age, housing in older facilities, access to outdoor areas, and multiple‐age stocking, have been linked to high Salmonella prevalence in commercial egg production operations. Housing systems for laying hens (including conventional cages, cage‐free options such as aviaries, and intermediate alternatives such as enriched colony cages) have been intensively investigated as potential Salmonella risk factors, but no definitive overall consensus has yet emerged from these studies. Some investigations have reported greater frequencies of Salmonella infection or environmental contamination in conventional cage‐based housing, but others have observed greater prevalence or transmission of Salmonella infection in cage‐free housing systems. In a large field survey, different housing systems did not significantly affect the environmental prevalence of Salmonellae, but distinctive Salmonella reservoirs and risk factors were identified for each system. Experimental infection studies have associated higher hen stocking density with increased susceptibility to both intestinal colonization and invasive infection by S. Enteritidis. Stress from overcrowding has been implicated in the suppression of immune responsiveness to Salmonella infection (Gast and Porter Jr., 2020).
5.4 Transmission
Molecular typing of isolates has demonstrated Salmonella dissemination from a breeding flock to multiple broiler flocks, and ultimately into the abattoir. Vertical transmission of Salmonellae to the progeny of infected breeding flocks can result from internal or external contamination of eggs. Egg shells can be contaminated with feces during oviposition. Salmonella penetration into or through shells and shell membranes can either directly transmit infection to developing embryos or allow exposure of chicks to infection when the shell structure is disrupted during hatching. S. Enteritidis can be deposited in the contents of eggs before oviposition. The resulting transovarian transmission of infection to progeny is an important aspect of S. Enteritidis epidemiology in chickens. The same Salmonella serovars responsible for mortality in naturally infected chicks or poults are often also found in their parent flocks. Vertical transmission from breeders has been identified as the principal route for dissemination of infection with host‐adapted S. Pullorum in chickens and has also been implicated in the introduction of S. Arizonae infection into turkey flocks. The Salmonella status of newly hatched chicks is a leading risk factor for infection in mature commercial layer and broiler flocks. Any Salmonellae carried in or on eggs can spread extensively in hatcheries. As chicks or poults pip through egg shells, Salmonellae are released into the air and circulated around hatching cabinets on contaminated fluff and other hatching debris. Samples of egg fragments, belting materials, and paper pads from broiler hatcheries have been Salmonella‐contaminated. Newly hatched birds, lacking protective intestinal microflora, are highly susceptible to intestinal colonization by Salmonellae. Chicks from uncontaminated eggs became infected with S. Typhimurium when hatched along with surface‐contaminated eggs.
Horizontal contact between newly hatched chicks played an important role in an outbreak of S. Pullorum infection in commercial broilers. Salmonella prevalence in broiler hatcheries is reportedly greater than at later production stages, and the serovars isolated from hatcheries sometimes correlate with those found on finished carcasses. Salmonella serovars carried from hatcheries by chicks often predominate in broiler flocks. Poultry environments are often implicated as principal sources of Salmonellae. Serovars present in broiler houses during the rearing period are likely to appear on processed carcasses. Litter contamination during production was reported as highly predictive of eventual carcass contamination. Studies in commercial laying flocks suggested that S. Enteritidis infection was more often acquired from farm environments than from breeders. Dust levels are also important risk factors for Salmonellae in poultry. S. Enteritidis persisted for at least 1 year in dust in an empty poultry house, even after cleaning and disinfection. In commercial laying houses, voided feces, manure belts, egg belts, and floors are recurrent areas of high Salmonella contamination. Horizontal transmission of Salmonella occurs both within and between flocks. Infection in previous flocks is a significant risk factor for Salmonella in both broilers and layers. Salmonella infection spreads via direct bird‐to‐bird contact or ingestion of contaminated feed, water, feces, or litter. S. Enteritidis was found in the internal organs of uninoculated laying hens housed in cages adjacent to orally inoculated birds. Circulation of personnel, equipment, aerosols, and dust throughout houses facilitates bacterial dissemination. Air samples collected both inside and outside of commercial laying houses were Salmonella‐positive. Airborne transmission of Salmonella infection has been reported, apparently mediated by contaminated dust. Levels of airborne Salmonellae in broiler houses have been linked to levels in litter. Infection can spread rapidly among chicks reared on litter (Gast and Porter Jr., 2020).
In human, poultry is considered a major reservoir for many Salmonella serovars, making salmonellosis a global issue. For example, in the United States of America, approximately more than 70% of human salmonellosis cases have been linked to the consumption of contaminated chicken, chicken products, or eggs. Salmonella enterica serovars Typhimurium (S. typhimurium) and Enteritidis are known to represent the main risk for public health. However, since 2018 there has been an increase in Salmonella enterica serovar Infantis (S. infantis) in poultry. A recent outbreak of food poisoning linked to fresh chicken meat contaminated with S. infantis, resulted in the infection of 129 people from 32 states, 25 hospitalizations, and one death reported in New York. In Europe, Salmonella is the second most-common cause of foodborne outbreaks, from which S. enteritidis and S. infantis have also been recently linked to frozen, raw, breaded chicken products that have caused almost 500 illnesses and one known death in 2020.
In poultry, Salmonella colonization of the gastrointestinal tract (GIT) can occur due to horizontal transmission when birds infected with Salmonella shed the bacteria in their feces and as a result, infect the environment and other closely housed birds. Young birds are mainly susceptible to Salmonella colonization by horizontal transmission at the hatcheries during feeding, handling, and transportation. Salmonella infection can also occur due to vertical transmission when Salmonella infects the ovaries of egg-laying hens. The infected hen then passes the contamination to the eggs before the formation of the shell, which results in infected progeny. Furthermore, mobile Salmonella can cause infection directly by ascending from the cloaca to the oviduct. Interestingly, Salmonella can colonize the GIT of birds without causing disease; hence, poultry can be infected with up to log 5 CFU Salmonella and be asymptomatic carriers.
After oral ingestion, Salmonella can easily thrive as an enteric pathogen due to its ability to persist in both acidic and basic environments, within a pH range of 4 to 9. Some Salmonella can grow at a pH as low as 3.7. In brief, upon oral ingestion, Salmonella will survive passage through the low-pH conditions of the gut. As it reaches the small intestine, the Salmonella bacterium adheres to and invades the intestinal epithelial cells. In the intestinal epithelia, Salmonella can be transported through the mucosa, chiefly via microfold cells (M cells), to gain access to the submucosa and underlying lymphoid tissue. Subsequently, macrophages within the underlying lymphoid tissue engulf Salmonella cells in an effort to eliminate the pathogen yet are unable to kill them due to the ability of the bacteria to interfere with phagosome-lysosome fusion. Additionally, other phagocytes, such as dendritic cells (DCs) and polymorphonuclear cells can also phagocytose Salmonella. Salmonella enterica uses many virulence factors to thrive within the host, but it specifically utilizes the type III secretion systems (T3SS) that are encoded in the Salmonella pathogenicity island 2 (SPI2) for its survival and intracellular replication within phagocytes. Within macrophages, Salmonella proliferates and is eventually disseminated. The typhoidal Salmonella serotypes can cause typhoid fever by disseminating from the intestinal mucosa and invading the bloodstream and distant organs, for example, the spleen, liver, and gallbladder. Non-typhoidal Salmonella infections, with serovars such as S. typhimurium and S. enteritidis, can be invasive or non-invasive. Non-typhoidal/non-invasive serotypes usually remain localized to the GIT, causing inflammation of the mucosa and secretory diarrhea (Acevedo-Villanueva, Akerele et al., 2021).
5.5 Environment factors
Feeds containing contaminated animal proteins, vegetable proteins, or cereals, or contaminated by vermin or wildlife, are potential sources of Salmonella in both chickens and turkeys. Chicks can be infected by very low levels of Salmonellae in feed. Salmonella contamination was found in 28% of finished feed samples from commercial broiler farms, although much lower frequencies of contamination are more common. Meal or mash feeds more often harbor Salmonellae than pelleted feeds. Salmonellae can survive for 2 years in inoculated feeds. Salmonella isolates from feedstuffs given to commercial poultry have been found to have genetic profiles which matched those of isolates from poultry meat and eggs produced by those flocks. Contaminated sources of drinking water can also transmit infection. The wide paratyphoid Salmonella host range creates numerous reservoirs of infection for poultry. Biologic vectors introduce, disseminate, and amplify Salmonellae in flocks. In Belgian egg‐laying farms, molecular fingerprinting linked S. Enteritidis isolates from farm environments, rats, mice, mites, flies, hens, and eggs. Insects and other invertebrates, including flies, darkling (litter) beetles, ground beetles, cockroaches, red mites, and centipedes can carry Salmonella organisms externally or internally. Salmonella infection has been transmitted by feeding contaminated flies or darkling beetles to chickens. Mice and rats are important vectors for S. Enteritidis in laying flocks. A study of commercial laying flocks in the United States reported an S. Enteritidis prevalence in mice from environmentally positive poultry houses which was 4 times that of negative houses. High rodent densities have been correlated with a greater likelihood of Salmonella infection in laying hens. A single rodent fecal pellet can contain 108 Salmonella cells. Wild birds and other wildlife are sometimes identified as Salmonella risk factors for poultry. Humans can also transmit Salmonella infection into poultry flocks (Gast and Porter Jr., 2020).
6. Pathogenesis
Although Salmonellae invade epithelial cells throughout the intestinal tract, the ceca and ileocecal junction are sites of particular affinity. After oral inoculation of chicks, S. Enteritidis adhered to epithelial cells at the tips of villi. Salmonella invasion changes the density and morphology of intestinal epithelial cells, affecting intestinal fluid and electrolyte regulation, and ultimately causing cell death and diarrhea. Oral inoculation of laying hens with S. Enteritidis produces inflammation of the epithelium and lamina propria of the ceca and colon caused by heterophilic infiltration. Epithelial invasion may also allow removal of Salmonellae through the basement membrane into the lamina propria by macrophages. The ability of Salmonellae to survive and multiply in internal organs, particularly within mononculear phagocytes of the liver and spleen, is a key element of their virulence. S. Pullorum is unable to multiply inside these cells in ducks, perhaps accounting for their resistance to pullorum disease. S. Enteritidis was recovered from several internal organs of laying hens within 1 hour after oral infection. S. Enteritidis numbers peaked at 24–36 hours after oral inoculation of chicks. The spleen may provide a protected site for intracellular Salmonella multiplication without exposure to host defense mechanisms. Many S. Enteritidis strains, as well as some strains of serovars such as S. Heidelberg and S. Typhimurium, are invasive for cells of the reproductive tract.
7. Clinical signs and pathology
7.1 Clinical signs
In general, paratyphoid Salmonella infections of poultry typically cause clinical signs only in very young chicks or poults. Salmonella contamination within eggs may lead to embryo mortality or rapid death among newly hatched birds before clinical signs are observed. Typical signs of severe Salmonella infection in chicks and poults include progressive lassitude and somnolence with closed eyes, drooping wings, ruffled feathers, shivering and huddling near heat sources, anorexia, emaciation, and profuse watery diarrhea (often resulting in dehydration and pasting of the vent area). Morbidity and mortality can be high during the first 2–3 weeks of life, with significant body weight loss or growth retardation, but signs of disease are infrequent in older birds. The course of illness is normally relatively brief in individual birds. Signs of severe Salmonella infection in young poultry are generally similar to those observed with other bacteria which cause acute septicemia. Although clinical disease is not characteristic of paratyphoid infections in mature poultry, some S. Enteritidis strains have caused anorexia, diarrhea, and reduced egg production in experimentally infected laying hens. Among other paratyphoid Salmonellae of note, S. Arizonae mostly affects turkey poults up to 4 weeks of age, causing relatively nonspecific clinical signs of depression, weakness, and diarrhea, with mortality approaching 50% in some instances. Other signs often associated with S. Arizonae infection in turkey poults include paralysis with torticollis and terminal opisthotonus. Infection of turkey breeding hens with S. Arizonae can decrease the production and hatchability of eggs. S. Pullorum infection causes egg‐transmitted disease in young chicks and turkey poults, often associated with white diarrhea and high mortality, but signs can be minimal with mortality peaking at 2–3 weeks after hatch. Blindness, labored breathing, and lameness caused by swelling of the hock joint are occasionally observed. Dead chicks may be found in the hatcher, but mortality is usually greatest after 10 days of age. Clinical signs, if any, include diarrhea with urate staining of the vent, decreased feed consumption, and huddling near heat sources. Adult birds are generally more resistant to infection and infected individuals are often asymptomatic, but they can transmit infection through eggs to hatchlings. S. Gallinarum infections of mature birds are sometimes responsible for substantial levels of morbidity and even mortality (Gast and Porter Jr., 2020).
7.2 Pathology
Severe outbreaks of Salmonella infection in young chicks or poults can involve rapidly developing septicemia with high mortality and few apparent lesions. Omphalitis and yolk sac infection are commonly associated with Salmonella infection of newly hatched poultry. The navel skin is often red or covered with a scab, and the yolk sac is typically filled with either blood or watery yellow fluid with flocculent material (Figure 7). When the course of disease is longer, enteritis with mucosal necrosis is observed (possibly with tan pseudomem-brane; Figure 8), along with white to yellow caseous exudate in the lumen of the ceca (“cecal cores”; Figure9). The liver is enlarged and often has pinpoint, pale to hemorrhagic foci of necrosis (Figure10). Splenic enlargement is also common. Kidneys may be enlarged and congested. Both the heart and liver are frequently covered with fibrin. Other occasionally observed lesions of paratyphoid Salmonella include accumulation of fibrin or fibrinopurulent exudate on the surfaces of the heart, liver, coelomic viscera, lung, and also within the eye (hypopyon) and wing or leg joints (arthritis).
Post-mortem lesions seen in salmonellosis may consist of dehydration, emaciation, an unresorbed or poorly resorbed yolk-sac, infection of the yolk-sac and necrotic debris in the yolk-sac, airsacculitis, splenomegaly, hepatomegaly, necrotic foci and petechiae in the liver and spleen, pseudomembranous perihepatitis and peritonitis, involving the serosal surfaces of the intestines, ovary and oviduct, enteritis, characterized by a watery intestinal contents and reddened areas of the mucosal surface of the duodenum, ileum and colon, typhlitis, with or without bloodstained or inspissated caecal cores, diarrhea, mild to severe mucopurulent or fibrinous pericarditis and dilatation of the pericardial sac by a thin bloodstained or a thick purulent fluid. Other pathological findings in 1- to 2-week-old broilers naturally infected with S. typhimurium were hypopyon, panophthalmitis and a purulent arthritis. Histopathological findings in hens infected with S. enteritidis PT4 were foci of necrosis and infiltration by heterophils and lymphocytes in the liver parenchyma and around the portal tracts, increased lymphoid tissue in the lamina propria and submucosa of the duodenum, ileum, caeca and colon, fusion and distortion of the intestinal villi and lymphocytic infiltration in the oviduct and in the tubules and around the larger collecting ducts in the. The caeca are the site within the intestinal tract of infected chickens that most commonly contain Salmonella. Examination of 12 different sites of the intestinal tract of chickens inoculated orally at 4 weeks of age with 108 S. typhimurium and S. infantis bacteria and examined at 0, 1, 2, 3, 6, 9, 13 and 17 days p.i. showed that 39.6% were positive by cloacal swab taken just prior to necropsy, 46.7% by caecal tonsil culture, 58.2% by cloacal-content culture and 85.2% by caecal content culture. There were no significant differences in localization between the two serovars of Salmonella. Salmonella was more frequently isolated from the caeca than from any other organs, tissues or other parts of the gastrointestinal tract of 6- month-old SPF Brown Leghorn cockerels infected orally with 1010 S. typhimurium (Poppe, 2000).
Gross lesions associated with S. Arizonae infection in turkey include omphalitis, retained yolk sacs, hepatosplenomegaly and white, caseous exudate in the ceca. Poults with neurological signs may have white (fibrinopurulent) exudate within the eye (hypopyon opthalmitis), and also on the meninges of the brain (meningoencephalitis) (Gast and Porter Jr., 2020).
8. Diagnosis
8.1 Isolation and identification of causative agent
Because clinical signs and gross lesions associated with Salmonella infection can resemble those caused by a variety of other bacteria, final diagnosis depends on the isolation and identification of causative organisms. Using conventional culture methods, this requires 48–96 hours (and even longer for some protocols). A concise summary of traditional methods for isolating Salmonella from poultry was provided previously. Numerous faster alternative strategies for detecting and identifying Salmonellae have been proposed and studied. Serologic detection of specific antibodies is sometimes employed as a rapid preliminary screening test to identify flocks that have been exposed to Salmonellae (Gast and Porter Jr., 2020).
8.2 Sample selection
Samples from a variety of sources, including tissues, eggs, voided feces, and poultry house environments, are collected and tested to identify Salmonella infection in flocks. The number of samples which must be processed to achieve a predetermined level of confidence of accurate detection is directly related to flock size and inversely related to the prevalence of infection. In very large flocks estimated to have low Salmonella prevalence, multiple samples are sometimes pooled together before culturing to improve the likelihood of detection within the limitations of existing laboratory resources.
Many Salmonella serovars are highly invasive and can spread systemically to numerous internal tissues, so a diversity of sites (including liver, spleen, ovary, oviduct, testes, yolk sac, heart, heart blood, kidney, gallbladder, pancreas, synovia, and eye) can provide samples for diagnostic culturing. Because lesions do not reliably indicate infected tissues, several different organs should be cultured from each bird (separately or together). Livers and spleens, as filtering organs, are most likely to be contaminated and thereby are the most effective culture targets for identifying infected birds. Egg culturing is often applied to assess the potential threat to public health posed by laying flocks infected with highly invasive serovars, particularly S. Enteritidis, which are deposited in the contents of eggs before oviposition.
Because paratyphoid Salmonella infections in poultry almost invariably involve intestinal tract colonization, samples of intestinal tissues and contents are frequently a focus for testing. In a diagnostic survey, Salmonellae were found exclusively in intestinal samples in 78% of chickens and 70% of turkeys. In experimentally infected laying hens, S. Enteritidis was recovered more often from the intestinal tract than from any other sampled tissue. The caudal ileum, ceca, cecal tonsils, and cecal contents are often recommended for Salmonella recovery, although no specific sample ensures detection of all infected birds. Cloacal swabs or fecal samples can provide sensitive indicators of persistent intestinal colonization in individual birds, but their diagnostic reliability is diminished by the intermittent shedding of Salmonellae in the feces of infected birds.
Fecal shedding of Salmonella into poultry house environments by infected birds makes culturing environmental samples a valuable diagnostic tool. Environmental samples also provide opportunities to monitor the introduction of Salmonellae into poultry houses by feed, vectors, personnel, and equipment. Sampling floor litter has sometimes provided a level of detection comparable with fecal samples. Dragging moistened gauze pads across floors in poultry houses has detected Salmonellae with high sensitivity. Swabs dragged through wet areas of manure are more productive than dry areas. Foot covers worn in poultry houses can also provide effective samples for detecting environmental Salmonellae. Nest boxes, egg belts, dropping belts or scrapers, fan blades, and dust are particularly productive sources for Salmonella sampling in laying houses. Dust samples may provide more consistent Salmonella detection than fecal samples, but combined testing of both optimizes detection sensitivity. Hatcher fluff and hatched eggshell membranes are frequently contaminated with Salmonellae, offering opportunities for early detection of infection. Air sampling detected Salmonella in both hatching cabinets and rooms housing infected chickens (Gast and Porter Jr., 2020).
8.3 Standard culture methods for Salmonella detection
Although diverse culture conditions are applied to isolate and identify Salmonellae, most standard methods follow a general scheme involving 4 principal stages. First, nonselective pre‐enrichment encourages the growth of very small numbers of Salmonellae or resuscitates injured cells. Pre‐enrichment is sometimes omitted when testing samples (such as intestinal contents or feces) with large numbers of competing organisms that might overgrow Salmonellae in nonselective media. Second, selective enrichment allows additional expansion of the Salmonella population while suppressing the growth of other organisms. Third, plating on selective agar media yields isolated colonies, each derived from a single cell. Nonselective agar plating media are also sometimes used with swabs from internal organs. This can be especially useful for detecting strains of S. Pullorum, which may grow slowly or produce very small colonies in the presence of some selective media ingredients. Fourth, colonies with appearances characteristic of Salmonellae are subjected to biochemical and serologic tests to confirm their genus and serovar identity. Virtually all proposed methods require the last 2 of these steps, but enrichment requirements vary according to the nature of the sample.
Internal organs (except for intestinal samples) from infected birds ordinarily contain relatively few competing organisms. Swab or loop samples taken from internal tissues are often transferred directly to plates of both selective and nonselective agar media, without broth enrichment. Excised tissue samples, and any samples derived from the intestinal tract, are generally transferred initially into selective enrichment broth.
Because fecal contamination may result in the presence of diverse flora, eggshells are usually sampled for Salmonella without pre‐enrichment (unless detecting the presence of other bacterial contaminants is also of interest). The surface of eggshells can be sampled by immersion or rinsing in broth media or the entire shell (including interior structures and shell membranes) can be sampled by aseptic breaking to release the contents followed by manual crushing and the addition of enrichment broth. Before culturing egg contents for contamination by Salmonellae, the exterior surface of shells must be disinfected to prevent the transfer of fecal contaminants to the contents during breaking.
Salmonella contaminants inside eggs are typically present at both very low prevalences and in very small numbers, so the entire liquid contents of 10–20 eggs are often pooled together for sampling to minimize demands on laboratory resources. Egg contents pools are usually incubated before further culturing to allow Salmonella populations to expand to consistently detectable levels. Supplementation of whole egg pools with iron can increase Salmonella multiplication during incubation. Pre‐enrichment of egg contents leads to more sensitive Salmonella detection than direct selective enrichment, perhaps by allowing expansion of small initial levels of bacteria before they are exposed to harsh selective enrichment conditions. Direct plating of incubated egg pools onto selective agar media reduces time, media, and labor requirements, but provides significantly poorer detection sensitivity.
Environmental samples are typically collected in sterile plastic bags and subsequently cultured by transfer into selective enrichment broth. Moistened gauze pads can be used to sample environmental surfaces or can be dragged across floor litter or dropping pits. Transporting environmental swab samples in double‐strength skim milk or buffered peptone water may improve Salmonella detection. Poultry feed should be tested by collecting several representative samples from each lot and transferring into selective enrichment broth. Pre‐enrichment of environmental and feed samples has not consistently improved Salmonella recovery (Gast and Porter Jr., 2020).
8.4 Culture media
A broad assortment of media has been developed and evaluated for isolating and identifying Salmonellae. Although some evidence suggests that proper selection of culture media is contingent on the type of sample being tested, several commercially available formulations have been consistently effective for a variety of applications. Suggested broth media to pre‐enrich samples for Salmonellae include trypticase soy broth and buffered peptone water. The selective broth media most often used for Salmonella detection in recent years are tetrathionate broth and Rappaport–Vassiliadis broth. Modified semi-solid Rappaport–Vassiliadis (MSRV) medium has sometimes performed as well as traditional broth media for selectively enriching Salmonella from poultry samples.
Numerous agar media are available for isolating Salmonellae. Among the most commonly used plating media are brilliant green, xylose‐lysine‐deoxycholate (XLD), xylose‐lactose‐tergitol 4 (XLT4), Rambach, bismuth sulfite, and Hektoen enteric agars. Brilliant green agar has been widely and successfully applied for Salmonella isolation from diverse poultry sources, including tissue, environmental, egg, feed, and air samples. XLT4 agar has also been effectively employed for detecting Salmonellae in poultry house environmental samples. Novobiocin addition to media improves Salmonella recovery by suppressing the growth of competitors such as Proteus. Samples should always be plated on 2 different agar media, preferably with dissimilar indicator systems for differentiating Salmonellae from other organisms.
Most culture media are incubated for 24 hours at 37°C. Shorter selective enrichment is usually inadequate to suppress competing microflora in heavily contaminated samples. Incubation of some selective enrichment media at elevated temperatures (41°C–43°C) has been recommended to restrict the growth of competing organisms, especially in intestinal samples or samples containing fecal material.
8.5 Confirmation of genus and serovar
Characteristic Salmonella colonies on selective agar plates must be tested further to confirm their genus identity and determine their serovar. Combined use of triple sugar iron agar and lysine iron agar provides an effective presumptive test for Salmonellae. The observed pattern of fermentation with a battery of 6 carbohydrates can provide further differentiation of Salmonella isolates from other organisms. The serogroup of isolates can be determined by agglutination tests with polyvalent antisera for somatic O antigens, and the serovar can then be determined by slide agglutination tests with monovalent antisera to specific O antigens and tube agglutination tests with antisera to flagellar H antigens. Molecular genetic Salmonella serotyping methods, such as intergenic sequence ribotyping, have become more widely utilized in recent years.
8.6 Rapid detection technologies
Obtaining Salmonella‐negative results using conventional culturing methods requires several days for most types of samples, and confirming positive results adds even more time. Considerably faster techniques are available and are increasingly becoming accepted as standard practices, although rapid methods have not altogether supplanted traditional culturing for most applications. Rapid methods typically reduce time requirements for testing by 1 or more days, and many are adaptable to some degree of automation. The principal limitations of rapid methods are related to cost and detection sensitivity. Rapid methods nearly always require at least 1 enrichment step to achieve detectable cell densities. Particularly high detection thresholds have been reported for nonmotile, slow‐growing strains of S. Pullorum. Most efforts to develop rapid Salmonella‐detection methods have centered around the use of specific antibodies or DNA probes.
Specific antibodies have been utilized in a variety of enzyme immunoassay (EIA) methods to detect Salmonella antigens. Polyclonal antibodies to LPS or flagella have detected Salmonellae in eggs, tissues, cloacal swabs, environmental drag swabs, litter, and feed. Likewise, assays using monoclonal antibodies to LPS, outer membrane proteins, or flagella have been applied to detect Salmonella (or specific serovars) in eggs, tissues, and environmental samples. Recent innovations in antibody‐based methods have attained sensitivities comparable with standard culture methods, although 1 or more initial enrichment steps are typically required to support expansion of the Salmonella population to detection threshold values of at least 105 cells/mL. Other antibody‐based assay formats have also been effectively applied for detecting Salmonellae in poultry samples. An important limitation on the usefulness of antibody‐based tests is their propensity to yield false‐positive results from competing flora which are able to grow in enrichment media.
Another application of antibodies for Salmonella detection is immunomagnetic separation (IMS), which employs small magnetic beads coated with specific antibodies to bind Salmonella target antigens in samples and remove them when a magnetic field is applied. IMS offers a faster alternative to broth enrichment for concentrating Salmonellae without adversely affecting sub-lethally injured cells. IMS concentration supported detection of small numbers of S. Enteritidis in pooled egg samples by both culturing and EIA. IMS concentration plus an EIA detected Salmonella from poultry environmental swabs with 98% of the sensitivity of traditional culturing, but required only 48 hours for testing.
An increasingly prominent approach to rapid testing for Salmonella is based on detecting genus‐specific or even serovar‐specific genetic sequences by hybridization of specific probes with target DNA extracted from samples. DNA hybridization typically detects Salmonellae at sensitivity thresholds similar to EIA, and thus usually requires 1 or more preliminary enrichment culturing steps. Moreover, DNA hybridization assays are procedurally complex and are often more expensive than other available methods. The development of PCR technology has allowed amplification of specific target segments of DNA, thereby enabling hybridization reactions with probes to detect Salmonella in tissues, environmental swabs, feces, and eggs with a high level of sensitivity. After enrichment culturing, PCR methods have detected initial contamination loads of fewer than 10 Salmonella cells in eggs and poultry environmental samples. Carefully chosen DNA probes can be used with PCR to detect Salmonellae with particular characteristics, such as genes for virulence factors, biochemical properties, or surface structures such as fimbria. A duplex PCR assay (specific to 2 different gene targets) was used to effectively differentiate S. Pullorum and S. Gallinarum isolates from other Salmonellae and also from each other. Multiplex PCR assays can simultaneously detect the presence of several serovars. Some PCR‐based tests are reportedly able to distinguish attenuated Salmonella vaccine strains from wild‐type field isolates.
8.7 Serologic diagnosis of infection
Specific antibodies to Salmonellae can be found in infected poultry with high sensitivity using diverse agglutination and EIA methods. Serologic testing has played an especially critical role in programs to control S. Pullorum‐Gallinarum infections in poultry breeding flocks of economically developed nations, such as the NPIP in the United States. These programs apply assays for serum antibodies to screen flocks for the presence of serologic reactors. Subsequent testing for the pathogen in internal organs of sampled birds provides confirmation of active infection and serves as the basis for removal of positive individuals or the depopulation of infected flocks.
Detectable serum antibody titers are often present long after clearance of all Salmonellae from tissues and cessation of fecal shedding. Because antibody tests only document prior Salmonella exposure, and do not provide unequivocal evidence of ongoing infection in flocks, positive serologic results must be followed by bacteriologic culturing for confirmation. Serology also yields positive results much later after infection than bacteriologic culturing. Other serologic testing limitations include subclinical infections which lead to fecal shedding without eliciting detectable antibody responses, immunologic unresponsiveness in very young birds, cross‐reactions between antibodies to antigenically related Salmonella serovars, and vaccine‐induced antibody responses which confound serologic differentiation of vaccinated and infected birds.
Agglutination tests have detected both natural and experimental infections of chickens with Salmonellae. Agglutination assays are performed on both whole blood and serum in plate, tube, and microwell formats. All of these tests rely on the ability of specific antibodies to visibly agglutinate killed whole Salmonella cells, which are stained (except in tube tests) to improve visualization of agglutination reactions.
Salmonella infections in poultry can also be detected using diverse EIA approaches. An international survey reported a high degree of correspondence in the performance of a wide assortment of EIA formats and antigens for detecting S. Enteritidis infections. EIA using LPS, flagella, or outer membrane protein antigens has identified chickens infected naturally or experimentally with S. Typhimurium, S. Enteritidis, or S. Pullorum. Screening for serum antibodies using a flagella‐based EIA was applied successfully for controlling S. Enteritidis in Dutch breeder flocks. By using very precisely defined antigens, EIA often achieves a high degree of specificity and thus produces fewer false‐positive cross‐reactions between serovars than agglutination reactions. Assays employing fimbrial antigens have shown especially high specificity for identifying S. Enteritidis infections in chickens. Antibodies deposited in egg yolks by infected hens offer uniquely convenient samples for Salmonella testing. A variety of assay formats have been applied to find anti-bodies to Salmonellae in eggs from naturally and experimentally infected chickens, correlating directly with the incidence of fecal shedding and organ invasion. Antibodies were detected by flagella‐based EIA in egg yolks from hens inoculated orally with as few as 103 S. Enteritidis cells. In both experimental infection studies and epidemiological field investigations, the detection of egg yolk antibodies to S. Enteritidis and the detection of the pathogen in feces or environmental swabs were similarly effective for predicting the production of contaminated eggs by infected hens (Gast and Porter Jr., 2020).
9. Immunity
The immune response of poultry to Salmonellae minimizes the duration and severity of infection and protects against reinfection. This response also serves as the basis for protecting birds against infection by vaccination and permits serologic detection of infected flocks. Administering immunosuppressive agents to chicks increased mortality associated with S. Typhimurium infection. Salmonella infection of chickens can cause lymphocyte depletion, atrophy of lymphoid organs, alteration of host immune signaling pathways, and immunosuppression, thereby facilitating the establishment of a persistent carrier state. The invasive behavior of S. Pullorum and S. Gallinarum may be facilitated by their lack of immune‐stimulating flagella, perhaps enabling them to avoid provoking strong inflammatory responses in infected birds. Salmonellae can elicit strong antibody responses in infected poultry. In a naturally infected broiler breeder flock, 70% of the birds were found to be positive for antibodies to S. Enteritidis. Experimental infection of chicks with S. Typhimurium induced strong IgG, IgA and IgM responses. When laying hens were orally infected with S. Enteritidis, serum antibodies were produced by most birds by 1week post-inoculation, reached peak values at 2 weeks, and remained at high levels for more than 6 months. Serologic positivity to Salmonella persisted among infected chickens throughout a 1‐year period. However, a highly virulent strain of S. Pullorum was found to elicit a lower serum antibody titer in broiler chickens than did a less virulent strain. S. Enteritidis infection of laying hens also induces antibody production by gastrointestinal lymphoid cells. Specific antibodies to S. Enteritidis have been detected in the yolks of eggs laid by infected hens, reaching peak levels several weeks after the serum antibody response. The progeny of immune breeding hens may acquire partially protective immunity via transfer of maternal antibodies in egg yolks.
Cell‐mediated immunity to Salmonellae is also important in poultry. Avian heterophils are phagocytic and bactericidal and may play a vital role in restricting organ invasion during early phases of Salmonella infection. A temporary decrease in T‐cell responsiveness to S. Pullorum at the onset of egg laying was observed to coincide with invasion of the pathogen to reproductive tracts of infected hens. Stimulating the immune responses of heterophils increased the resistance of chicks to S. Enteritidis colonization. Cytokines produced by sensitized T lymphocytes expand the pool of circulating phagocytic heterophils and recruit them to the site of infection. Various intestinal cytokines and antimicrobial peptides are sequentially expressed during the course of Salmonella infection in chickens. S. Enteritidis infection in chicks induced the expression of chemokines which recruit macrophages and monocytes. Cytokine and chemokine expression profiles have been observed to vary for different Salmonella serovars.
Both humoral and cell‐mediated immune responses appear to play important roles in protecting poultry against Salmonella infection. The timing of clearance of S. Typhimurium infection in chickens correlated with the emergence of strong antigen‐specific cellular and humoral immune responses. Likewise, a decline in S. Enteritidis isolation from reproductive tissues of laying hens during the second week of infection was associated with the proliferation of both T and B lymphocytes. Both the opsonic activity of specific antibodies and the phagocytic and lytic activity of cellular effectors may be necessary for the full expression of immunity. In addition to antigen‐specific adaptive immune responses, innate host phagocytic capabilities also contribute significantly to resistance during the early stages of infection by Salmonellae. Chicken macrophages can internalize higher numbers of S. Enteritidis cells and clear intracellular Salmonellae more rapidly than lymphocytes. Innate responses are especially critical in newly hatched poultry which are immunologically immature and unable to mount fully protective adaptive immune responses to infection. Selection for genetically based differences in the innate resistance or immunity of lines of chickens to Salmonella infection has been considered as a potential protective strategy for flocks. Chicks from distinct lines can vary significantly in Salmonella‐associated mortality. Different incidences of fecal shedding, organ invasion, and egg contamination have been observed for lines of mature chickens infected with S. Enteritidis. Lines of chickens differing in Salmonella resistance have differed in T cell responses and the expression of cytokines, antimicrobial peptides, and pathogen‐specific cellular receptors. However, effective selection for Salmonella‐resistant lines of chickens is complicated by negative genetic correlations between resistance in chicks and adults and between resistance traits and production traits (Gast and Porter Jr., 2020).
10. Treatment
The efficacy and wisdom of medicating with antibiotics to prevent or treat Salmonella infections in poultry have been debated for many years. Antibiotics have a long history of widespread utilization in poultry at both therapeutic and subtherapeutic (growth‐promoting) levels. Their usefulness for these purposes has been extensively documented in both experimental and commercial settings. A variety of antibiotics have demonstrated either prophylactic or therapeutic activity against Salmonellae in poultry, in some instances leading to decreased fecal shedding when used as feed additives. Antibiotics were employed effectively for S. Enteritidis control in broiler and broiler breeder flocks in Northern Ireland. Treatment with a fluoroquinolone antibiotic, followed by provision of a competitive exclusion culture to restore protective normal microflora, has reduced fecal shedding of S. Enteritidis in broiler breeders, egg‐type pullets, and molted laying hens. In ovo administration of gentamicin controlled Salmonella infection without affecting the viability of competitive exclusion cultures given to the hatched chicks. However, current control practices for poultry salmonellosis in many nations no longer regularly rely on antibiotics because of both inconsistent performance of these drugs in eliminating Salmonella colonization and concerns that indiscriminate veterinary and agricultural use could promote microbial resistance. Limited efficacy of antibiotics for controlling Salmonella infections in poultry has been documented on numerous occasions. In some instances, antibiotic administration has actually increased susceptibility to Salmonella infection, perhaps by suppressing the growth of other competitive or inhibitory microflora. Discontinuing antimicrobial use for growth promotion in Denmark was followed by decreased Salmonella prevalence in broilers. Both therapeutic and subtherapeutic antibiotic administration can select for drug‐resistant strains of Salmonellae, thereby imperiling the medicinal usefulness of those (and related) agents for animals and humans. Drug resistance determinants can accumulate over time in Salmonellae present in poultry flocks. Very high incidences of antibiotic resistance have been reported among Salmonella isolates from both poultry production facilities and poultry products, with a large proportion of these strains often displaying resistance to multiple antimicrobials (Gast and Porter Jr., 2020).
Antibiotics: Choose antibiotics that have an antibacterial spectrum on Salmonella spp… There are some suggestive products;
- Injectable solution:
Vime Floro FDP: 1ml/5 kgs body weight, once a day in 4-5 consecutive days
Tylovet: 1ml/5 kgs bodyweight conce a day in 4-5 consecutive days
Vimekat: 1ml/5 kgs bodyweight, once a day in 2-3 consecutive days
- Oral administration:
Vimenro 200: 1ml/10-15kgs bodyweight or 1ml/1-1.5 liters of drinking water) apply in 5-7 consecutive days
Elecamin: 2ml/1liter of drinking water, apply in 5-7 consecutive days
11. Control and prevention
The diversity of sources for Salmonella introduction into flocks or houses complicates the identification of critical control points for preventing infection of poultry. Because intervention options are not always individually effective, successful prevention and control programs must involve coordinated and sustained implementation of diverse risk reduction practices throughout the production continuum (Hossain, Attia et al., 2021). Financial analysis has indicated that the costs of intensive Salmonella control efforts in poultry are justified by even greater savings in public health costs. Eggs and chicks (or poults) should be obtained only from demonstrably Salmonella‐free breeding flocks. Hatching eggs should be properly disinfected and hatched under stringent sanitation standards. Poultry houses should be thoroughly cleaned and disinfected by recommended procedures between flocks. Rodent and insect control measures should be incorporated into house design and management and verified by periodic testing. Rigidly enforced biosecurity practices should be implemented to restrict movement of personnel and equipment onto poultry housing premises and between houses. Only pelleted feed or feed containing no animal protein should be used. Water provided to poultry should come only from sources treated to ensure cleanliness. Treatments such as gastrointestinal colonization control or vaccination can be applied to reduce the susceptibility of birds to infection. Finally, the Salmonella status of poultry and their environment should be monitored by periodic testing to verify the effectiveness of risk reduction practices. Multifaceted risk reduction programs in breeding and laying flocks have been internationally associated with significant reductions in both the prevalence of S. Enteritidis infection in poultry and egg‐association human illness.
11.1 Gastrointestinal colonization control
Newly hatched chicks and poults are highly susceptible to Salmonella infection, but quickly become more resistant as they acquire protective intestinal microflora from their environment. The ability of the normal bacterial flora of the gastrointestinal tract to inhibit colonization by pathogens is the basis for a diverse group of treatments often referred to collectively as competitive exclusion (CE). Defined or undefined CE cultures are administered to poultry to diminish Salmonella colonization. Various nonmicrobial manipulations of gastrointestinal biochemistry have also been explored as colonization control options. CE treatment reduces the incidence and magnitude of Salmonella colonization in poultry, but rarely prevents it altogether. Accordingly, CE treatment can make a valuable contribution toward controlling Salmonellae, but it cannot entirely supplant comprehensive risk reduction programs. Competitive exclusion treatment with intestinal or fecal material from mature birds or undefined anaerobic cultures derived from such material has diminished both intestinal colonization and subsequent invasion to internal tissues by Salmonellae in both chickens and turkeys. In commercial broiler flocks, CE treatment significantly reduced the incidence of Salmonellae both in live birds and on carcasses. CE administration to egg‐type pullets before transfer into a contaminated laying house reduced subsequent Salmonella isolation from fecal and environmental samples. Treatment with CE cultures has sometimes enhanced the clearance of concurrent or preexisting Salmonella infections. Only live CE preparations exert protective effects, but their efficacy can be maintained by continuous flow culturing. CE cultures are effective when administered via oral gavage, spraying, or addition to drinking water. The protective efficacy of CE can be overcome by severe Salmonella challenges. Disruption of the normal intestinal microflora by feed or water deprivation, or by antibiotic administration, can interfere with the activities of CE cultures. Because CE cultures are most effective when administered before exposure to pathogens, infection during hatching can compromise the value of subsequent treatment. Individual CE preparations may not be equally protective in different avian species. Efforts to identify the microflora constituents responsible for CE effects have reported that cultures of diverse bacterial genera, including Lactobacillus, Bifidobacterium, Bacillus, Escherichia, Pediococcus, and Streptococcus, and the yeast Saccharomyces, exhibit protective (probiotic) activity against Salmonellae in chickens. Defined mixtures of microorganisms potentially perform with greater consistency and more assurance of safety than undefined cultures of unknown organisms. The protective efficacy of defined CE preparations is often higher for mixtures of greater diversity or complexity. Protection by CE cultures has been attributed to direct steric interference with Salmonella attachment to the intestinal epithelium, altered intestinal permeability to pathogens, or inhibition of intestinal Salmonella growth via lowered pH. Some probiotic cultures may also influence gene expression by Salmonellae or modulate the host immune response to infection. Diverse “prebiotic” additives either directly inhibit pathogen colonization or support the growth of protective microflora. Feed or water supplementation with various complex carbohydrates (including chitosan, mannanoligosaccharide, and fructooligosaccharide) has reduced crop or cecal colonization of chickens by Salmonellae. Supplementing feed with medium‐chain fatty acids (formic, propionic, butyric, caprylic, or caproic acids), or providing feedstuffs which are easily fermented to yield these acids, has been associated with lower frequencies of Salmonella isolation. Plant‐derived antimicrobial compounds such as trans‐cinnamaldehyde have also demonstrated protective efficacy as feed additives (Gast and Porter Jr., 2020).
11.2 Vaccination
Administration of either killed or live vaccine preparations can significantly reduce the susceptibility of poultry to Salmonella infection. Vaccination of laying flocks with several different combinations of killed and live vaccines all reduced the frequency of egg contamination with S. Enteritidis, although a lesser effect was observed on fecal shedding and poultry house environmental contamination. Decreased incidences of human S. Enteritidis infections have followed the widespread implementation of vaccination programs for egg‐laying hens. Neither type of vaccine has consistently provided an impenetrable barrier against infection, especially when high Salmonella challenge doses are involved. Feed or water deprivation and environmental stresses such as heat can compromise vaccine efficacy. Vaccination is often unable to confer protection against heterologous serovars. Like competitive exclusion, vaccination is most effectively used as a component within comprehensive risk reduction programs. The emergence of S. Enteritidis as an egg‐transmitted source of human illness generated renewed interest in killed vaccines (bacterins) for poultry. Killed vaccines have been associated with decreased incidences of S. Enteritidis infection in Dutch broiler breeder flocks and S. Enteritidis contamination in eggs from Japanese laying flocks. Subcutaneous or intramuscular vaccination of laying hens with adjuvanted bacterins induces long‐lasting antibody responses and has significantly reduced S. Enteritidis isolation from feces, internal tissues, and eggs following subsequent oral challenge. Consistently negative S. Enteritidis testing results were obtained from a vaccinated laying flock transferred into previously contaminated facilities. Bacterin administration to laying hens moderated the effects of induced molting by feed restriction on fecal shedding of S. Enteritidis. Progeny of breeding flocks vaccinated with Salmonella bacterins may display some degree of maternally acquired protective immunity to infection. Multivalent bacterins, prepared from a mixture of serovars or strains, can provide an expanded spectrum of protection. Immunization of chickens with subunit vaccines composed of outer‐membrane, fimbrial, or flagellar proteins has conferred significant protection against S. Enteritidis colonization. Bacterins which highly express these immunogenic surface components have been shown to have enhanced protective capabilities. Live attenuated vaccines must persist in tissues long enough to induce protective immune responses but should be avirulent and cleared from vaccinated birds within a few weeks of administration. A wide variety of Salmonella vaccine strains have been evaluated for protective efficacy in poultry, often incorporating multiple deletion mutations to ensure irreversible attenuation. Mutant strains unable to synthesize or utilize essential metabolites, produce flagella or fimbria, express virulence‐related proteins or lipopolysaccharides, or multiply at poultry body temperatures have all reduced Salmonella colonization after administration to chicks or poults by spraying or addition to drinking water. Recombinant vaccine strains can express molecules with adjuvant properties to enhance immunogenicity. Nonflagellated mutants may elicit protective immunity without preventing serologic detection of paratyphoid‐infected flocks. Live vaccine strains have been reported to enhance the innate immune response and to induce both humoral and cellular adaptive immune responses. Attenuated vaccines have been useful for protecting hens against increased susceptibility to S. Enteritidis infection following induced molting by feed deprivation and for enhancing protection by maternal antibodies in chicks from bacterin‐vaccinated hens. Evidence for cross‐protection by live vaccine strains against other Salmonella serovars has been inconsistent. Protection against challenge with antigenically unrelated Salmonella strains has been attributed to a combination of immunological and competitive exclusion mechanisms. The commercially available “rough” 9R strain of S. Gallinarum has been widely and successfully used for many years as a vaccine to control the incidence of fowl typhoid in regions where this disease is endemic. More recently, S. Gallinarum attenuated deletion mutants and “ghosts” (cell envelopes after expulsion of their cytoplasmic contents) have also been developed and demonstrated to have protective efficacy against infection. Live Salmonella vaccines have sometimes been associated with more complete protection of poultry, perhaps because of more persistent presentation of relevant antigens to the host immune system or because these antigens are adversely affected during the preparation of killed vaccines. Bacterins may also fail to fully elicit the cell‐mediated portion of the protective immune response. However, killed vaccines frequently stimulate protection of greater duration. Protection against infection following the combined application of both live and killed vaccines has often exceeded the performance of either product administered separately. Prophylactic administration of cellular lymphokines from immunized chickens can protect chicks against organ invasion following subsequent Salmonella challenge. This effect is associated with increased phagocytosis and killing of Salmonella by avian heterophils, but it may be of relatively transient duration (Gast and Porter Jr., 2020).
12. References
Acevedo-Villanueva, K. Y., et al. 2021. A Novel Approach against Salmonella: A Review of Polymeric Nanoparticle Vaccines for Broilers and Layers. Vaccines. 9:1041
Brownell, J.R., Sadler, W.W. and Fanelli, M.J. (1970) Role of bursa of Fabricius in chicken resistance to Salmonella typhimurium. Avian Diseases 14, 142–152.
Brownell, J.R., Sadler, W.W. and Fanelli, M.J. (1970) Role of bursa of Fabricius in chicken resistance to Salmonella typhimurium. Avian Diseases 14, 142–152
Chart, H., Rowe, B., Baskerville, A., and Humphrey, T.J. (1990). Serological response of chickens to Salmonella enteritidis infection. Epidemiology and Infection 104, 63–71.
Fanelli, M.J., Sadler, W.W., Franti, C.E. and Brownell, J.R. (1971) Localization of Salmonellae within the intestinal tract of chickens. Avian Diseases 15, 366–375.
Garai, P., et al. 2012. Salmonella enterica serovars Typhimurium and Typhi as model organisms. Virulence. 3:377-388
Gast, R. K. and R. E. Porter Jr. (2020). Salmonella Infections. Diseases of Poultry: 717-753.
Hafez, H. M. 2001. Salmonella infections in poultry: diagnosis and control. Periodicum Biologorum. 103:103 114
Henderson, S.C., D.I. Bounous, and M.D. Lee. 1999. Early events in the pathogenesis of avian salmonellosis. Infect Immun. 67:3580–3586.
Hitchcock, P.J. and Brown, T.M. (1983) Morphological heterogeneity among Salmonella lipopolysaccharide chemotypes in silver-stained polyacrylamide gels. Journal of Bacteriology 154, 269–277.
Hossain, M. J., et al. 2021. Zoonotic Significance and Antimicrobial Resistance in Salmonella in Poultry in Bangladesh for the Period of 2011–2021. Zoonotic Diseases. 1:3-24
Lissner, C.R., Swanson, R.N. and O’Brien, A.D. (1983) Genetic control of the innate resistance of mice to Salmonella typhimurium: expression of the Ity gene in peritoneal and splenic macrophages isolated in vitro. Journal of Immunology 131, 3006–3013.
Otomo, Y., K. Abe, K. Odagiri, A. Shiroto, K. Takatori, and Y. Hara‐Kudo. 2007. Detection of Salmonella in spent hens and eggs associated with foodborne infections. Avian Dis. 51:578–583.
Padron, M.N. (1990) Salmonella typhimurium outbreak in broiler chicken flocks in Mexico. Avian Diseases 34, 221–223.
Phillips, R.A. and H.M. Opitz. 1995. Pathogenicity and persistence of Salmonella enteritidis and egg contamination in normal and infectious bursal disease virus‐infected leghorn chicks. Avian Dis. 39:778–787.Poppe, C. 2000. Salmonella infections in the domestic fowl. Salmonella in domestic animals. 107-132
Revolledo, L. (2012). Avian Salmonellosis, vaccines and immune mechanisms of protection: present and future perspectives.
Schaaf, J. (1936) Die Salmonellose (infektiöse Enteritis, Paratyphose) des Geflügels, ihre Bedeutung und Bekämpfung. Zeitschrift für Infektionskrankheiten, Parasitäre Krankheiten und Hygiene der Haustiere 49, 322–332.
Skov, M.N., N.C. Feld, B. Carstensen, and M. Madsen. 2002. The serologic response to Salmonella enteritidis and Salmonella typhimurium in experimentally infected chickens, followed by an indirect lipopolysaccharide enzyme linked immunosorbent assay and bacteriologic examinations through a one‐ year period. Avian Dis. 46:265–273.
Sumner, J., G. Raven, and R. Givney. 2004. Have changes to meat and poultry food safety regulation in Australia affected the prevalence of Salmonella or of salmonellosis? Int J Food Microbiol. 92:199–205.
Wesley, I.V. and W.T. Muraoka. 2011. Time of entry of Salmonella and Campylobacter into the turkey brooder house. Food Bioprocess Technol 4:616–623.
WHO (2010) “Laboratory Protocol”. In: Isolation of Salmonella spp. From Food and Animal Faeces, 5th Ed. June 2010. Pages 4-8,13.
Wigley, P., A. Berchieri, Jr., K.L. Page, A.L. Smith, and P.A. Barrow. 2001. Salmonella enterica serovar Pullorum persists in splenic macrophages and in the reproductive tract during persistent, disease‐free carriage in chickens. Infect Immun. 69:7873–7879.
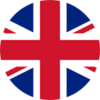