Nguyen Pham Thao Nhi, Tran Duy Thanh, Pham Trang Thanh Nguyen,
Nguyen Khanh Thuan, Nguyen Phuc Khanh, Nguyen Thanh Lam*
1. Introduction
African swine fever (ASF) is a fatal viral disease of pigs, affecting domestic pigs and wild boar of all ages without sex predilections. Depending on virus strain and immunological status of the animal, infection can lead to a wide range of clinical presentations varying from per-acute to chronic disease, including apparently asymptomatic courses. Infection with virulent strains typically causes per-acute to acute lethal ASF with signs including sudden death, high fever, hemorrhages in the skin and internal organs. The animals usually die within three to ten days after infection and the case fatality rate can reach 90% or more (Sánchez-Vizcaíno et al., 2015).
2. History
African swine fever was first described in 1921 in Kenya when the virus was transmitted from wild African suids to domestic pigs, causing a disease with 100% mortality. Since this discovery, ASF virus has left Africa on three occasions. The first incursion occurred in 1957 from Angola to Lisbon. The second (1960) was from Africa to Lisbon and then to Spain and other European countries, including France (1964), Italy (1967, 1969), the island of Sardinia (1978), Malta (1978), Belgium (1985), and the Netherlands (1986). From Europe, ASF virus spread to Latin America, including Cuba (1971, 1980), Brazil (1978), the Dominican Republic (1978), and Haiti (1979). Subsequently ASF virus was eradicated from all of these countries, except Portugal and Spain, where it remained endemic until 1995, and Sardinia, where it remains endemic today. A third ASF virus incursion from Africa occurred in 2007, when it entered the Caucasian region and spread to the Russian Federation (2007), Ukraine (2012), Belarus (2013), reaching the Baltic regions and Poland in 2014, and spreading westward to Central and Western Europe in 2017 and 2018. In August 2018 ASF was reported for the first time in China. All of these countries remain infected with ASF virus at present. The current ASF virus epidemiological situation poses a serious threat to animal health, pig production, and therefore the economies of affected and neighboring countries. ASF virus remains endemic in many sub‐Saharan countries on the African continent (Sánchez‐Vizcaíno et al., 2019).
3. Geographic distribution
African swine fever is currently widespread in sub-Saharan Africa, Eastern Europe and the Caucasus and the Italian island of Sardinia. With the increased circulation of ASF, there is growing global concern that the virus will spread further into other parts of the planet. Any country with a pig sector is at risk, and history has shown that the disease can jump thousands of kilometres into previously free countries, mostly via meat arriving aboard aircraft and ships and then incorrectly disposed of, or meat carried by individual travelers. Of particular concern is the potential spread into East Asia. With China relying heavily on the pork industry and owning almost half of the world’s domestic pigs, an ASF epidemic lead to catastrophic impact on trade and pig production, with serious implications for global food security. Official information on the status and dates of ASF outbreaks can be obtained from the World Animal Health Information System (WAHIS) at the World Organisation for Animal Health (OIE).
infected with ASF virus at present. The current ASF virus epidemiological situation poses a serious threat to animal health, pig production, and therefore the economies of affected and neighboring countries. ASF virus remains endemic in many sub‐Saharan countries on the African continent (Sánchez‐Vizcaíno et al., 2019).
3. Geographic distribution
African swine fever is currently widespread in sub-Saharan Africa, Eastern Europe and the Caucasus and the Italian island of Sardinia. With the increased circulation of ASF, there is growing global concern that the virus will spread further into other parts of the planet. Any country with a pig sector is at risk, and history has shown that the disease can jump thousands of kilometres into previously free countries, mostly via meat arriving aboard aircraft and ships and then incorrectly disposed of, or meat carried by individual travelers. Of particular concern is the potential spread into East Asia. With China relying heavily on the pork industry and owning almost half of the world’s domestic pigs, an ASF epidemic lead to catastrophic impact on trade and pig production, with serious implications for global food security. Official information on the status and dates of ASF outbreaks can be obtained from the World Animal Health Information System (WAHIS) at the World Organisation for Animal Health (OIE).
infected with ASF virus at present. The current ASF virus epidemiological situation poses a serious threat to animal health, pig production, and therefore the economies of affected and neighboring countries. ASF virus remains endemic in many sub‐Saharan countries on the African continent (Sánchez‐Vizcaíno et al., 2019).
3. Geographic distribution
African swine fever is currently widespread in sub-Saharan Africa, Eastern Europe and the Caucasus and the Italian island of Sardinia. With the increased circulation of ASF, there is growing global concern that the virus will spread further into other parts of the planet. Any country with a pig sector is at risk, and history has shown that the disease can jump thousands of kilometres into previously free countries, mostly via meat arriving aboard aircraft and ships and then incorrectly disposed of, or meat carried by individual travelers. Of particular concern is the potential spread into East Asia. With China relying heavily on the pork industry and owning almost half of the world’s domestic pigs, an ASF epidemic lead to catastrophic impact on trade and pig production, with serious implications for global food security. Official information on the status and dates of ASF outbreaks can be obtained from the World Animal Health Information System (WAHIS) at the World Organisation for Animal Health (OIE).
indicated by arrows and numbers. 1. In blue, the spread of genotype I virus from West Africa to Portugal in 1957 and 1960 is shown; this was thought to involve the
feeding to pigs of infected meat introduced from aircraft. 2. From the Iberian Peninsula, the virus spread to other European countries and to South America and the Caribbean. These outbreaks were eradicated by the mid-1990s, except from Sardinia, where the disease remains endemic. 3. In 2007, ASF virus genotype II spread from the east coast of Africa to Georgia in the Transcaucasus region, thought to be introduced through infected meat eaten by pigs from shipping near the Black Sea port of Poti. 4. From Georgia, the virus spread to neighbouring countries, including the Russian Federation and to eastern European countries, including a number in the EU. In 2018 there was further westward spread in Poland and to Belgium. 5. In 2018, ASF virus genotype II spread from Russia or Europe to China, rapidly spreading to many provinces and threatening further spread in the region. In 2019, the first outbreaks in Mongolia and Vietnam were reported. Outbreaks of ASF in domestic pigs reported to the OIE during 2018 are shown as red dots, and detections in wild boar as purple dots. African countries that reported the disease from 2007 to 2016 are shown in yellow, and those that have reported ASF since 2017 are shown in purple. Countries where the disease is restricted to certain zones are shown in turquoise (Dixon et al., 2019b).
4. Aetiology
4.1 Virus characteristics
ASF virus is a large, icosahedral, linear double‐stranded DNA virus and the only member of the family Asfarviridae, genus Asfivirus. The virion is composed of four concentric layers and an external hexagonal membrane (Figure 3) acquired by budding through the cell plasma membrane. ASF virus replication occurs mainly in the cytoplasm of infected macrophages, though an early stage of replication has also been described in the nucleus.
The viral genome varies in length from 170 to 193 kb and contains 150–167 open reading frames. The genome consists of a conserved central region of approximately 125 kb and two variable ends containing five multigene families (MGFs). Deletions and insertions of copied regions as long as 20 kb occur within the MGF genes, suggesting that these regions may help generate antigenic variability and therefore help ASF virus evade the host immune system (Sánchez‐Vizcaíno et al., 2019).
This virion atlas (Figure 4) provides the distribution of 40 viral proteins in the five virus structural domains. In this model, most of the detected proteins are localized at the DNA-containing nucleoid, including the major DNA-binding proteins pA104R and p10 (pK78R), whose localization has been determined by immune-electron microscopy, along with a set of putative transcription factors and viral enzymes involved in mRNA transcription and modification. It is known that ASF virus entry leads to the delivery of naked cores into the cytoplasm. Therefore, it seems reasonable to hypothesize that this transcriptional machinery is located at the viral nucleoid in order to ensure that early virus genes are properly transcribed and modified for subsequent translation. In this connection, vaccinia virus enzymes involved in early transcription are known to be associated with the viral core. Similarly, the viral dUTPase (pE165R) along with the set of three enzymes involved in DNA repair can also be tentatively located in this viral domain on the basis of its putative function. The localization of the viral A/P endonuclease (pE296R) at the nucleoid supports this assumption. Finally, the viral IAP homologue (pA224L) has also been located at the virus nucleoid (Alejo et al., 2018).
The core shell is essentially composed of the mature products derived from the proteolytic processing of polyproteins pp220 (ORF CP2475L) and pp62 (CP530R), which represent about one-third of the total virion mass, and the viral protease. The protein icosahedral capsid consists of the major capsid component p72 and the minor capsid protein p49, both of which are involved in the assembly of this domain, as well as the protein pE120R, which mediates the intracellular virus transport.
At least seven known membrane viral proteins (p17 [pD117L], pE183L, p12 [pO61R], p22 [pKP177L], pH108R, pE199L, and pE248R) localize at the inner envelope, while only one viral protein, the CD2 homologue pEP402R, has been unambiguously located at the outermost membrane. A possible reason for this uneven distribution is the pivotal role of the inner envelope in both the assembly and the entry of the ASF virus particle. Thus, the inner envelope should contain the proteins needed to transform and stabilize an endoplasmic reticulum-derived membrane into a viral membrane. Also, the inner membrane acts as a scaffold to support the multiple interactions required to assemble the outer capsid and the internal core shell. Finally, this membrane contains the fusion machinery required for the cytoplasmic core delivery that follows virus entry, which in the case of the related vaccinia virus includes up to 12 different polypeptides. At variance with many other enveloped viruses, the budding process of ASF virus is not concomitant with the assembly of a mature infectious particle. Indeed, the intracellular mature particles produced at the virus factories are infectious. This could explain, at least partly, the minor presence of viral proteins in the outer envelope. Despite this, it will be highly relevant to identify in future, specifically targeted studies the components of the outer viral membrane. These will most probably be involved in virus exit and attachment of the extracellular virions to the host cell, hence playing crucial roles in virus dissemination and pathogenesis (Alejo et al., 2018).
Resistance to physical and chemical action of ASF virus
Temperature: | Highly resistant to low temperatures. Heat inactivated by 56°C/70 minutes; 60°C/20 minutes. |
pH: | Inactivated by pH 11.5 in serum-free medium. Serum increases the resistance of the virus, e.g. at pH 13.4 – resistance lasts up to 21 hours without serum, and 7 days with serum. |
Chemicals/disinfectants: | Susceptible to ether and chloroform. Inactivated by 8/1000 sodium hydroxide (30 minutes), hypochlorites – between 0.03% and 0.5% chlorine (30 minutes), 3/1000 formalin (30 minutes), 3% ortho-phenylphenol (30 minutes) and iodine compounds. Note: disinfectant activity may vary depending on the pH, time of storage and organic content. |
Survival: | Remains viable for long periods in blood, faeces and tissues; especially infected, uncooked or undercooked pork products. Can multiply in vectors (Ornithodoros sp.). |
4.2 Classification
The species ASF virus was once included in the family Iridoviridae, but is now assigned to the genus Asfivirus, family Asfarviridae (Figure 6). The unclassified faustoviruses, kaumoebavirus and Pacmanvirus, have about 30 genes related to those of ASF virus, but their genomes are considerably larger (about 400 kbp compared to 170–194 kbp).
Different genomic regions have been targeted to detect ASFV phylogenetic relationships with different levels of precision. The current approach for investigating the molecular epidemiology of ASF is through sequencing of the C-terminal end of p72 (B646L) gene encoding the p72 major capsid protein in order to determine the viral genotype. So far, 24 ASFV p72 genotypes (I–XXIV) have been identified (Figure 7). Further discrimination into subgroups of closely related viruses is usually conducted by sequence analysis of the tandem repeat sequences (TRS) located in the central variable region (CVR) within the B602L gene and the intergenic region between the I73R and I329L genes. Several other genomic regions such as the E183L encoding the p54 protein, the CP204L encoding the p30 protein, and the EP402R gene encoding the CD2v protein, have proved to be useful tools for molecular epidemiological and virus spread investigations
4.3 Pathogenicity
Viral entry mechanisms
The ASF virus infectious cycle starts with the viral adsorption and entry into the host cell. Early studies on ASF virus entry characterized this event as a low pH- and temperature-dependent process consistent with saturable and specific receptor-mediated endocytosis in Vero cells and porcine macrophages. However, the receptor(s) for the virus still remain unknown. The limited cell tropism of ASF virus suggests that a macrophage-specific receptor is required for infection. Successful infection of porcine macrophages and monocytes by ASF virus correlates to the expression of the CD163 scavenger receptor, a hallmark of macrophage maturation. It was previously suggested as a potential virus receptor, as monoclonal antibodies against this molecule were able to block infection of primary alveolar macrophages. However, more recent studies have demonstrated that CD163 is not necessary for infection with the Georgia 2007/1 virus isolates. Gene-edited pigs possessing a complete knockout of CD163 produced using the CRISPR/Cas9 system showed no differences in clinical signs, mortality, pathology, or viremia. One conclusion from these studies was that CD163 may be necessary but insufficient for infection, suggesting that other macrophage surface proteins may participate in the infection process.
While there is support for receptor-dependent mechanisms of viral entry, such as clathrin-mediated dynamin-dependent endocytosis, there is also evidence that ASF virus exploits other mechanisms, such as phagocytosis and macropinocytosis. Also, cholesterol is required for a successful entry. These mechanisms occur both in the macrophage target cell and in Vero cells using viral isolates adapted to this cell line.
In addition, some ASF virus proteins are involved in the entry mechanism such as p30, important for viral internalization, while other proteins such as p12 and p54 have been identified as potential viral attachment proteins (Galindo and Alonso, 2017).
ASF virus enters the endosomal pathway
ASF virus infection by either pathway of entry should finally reach the endocytic pathway. Once the virus has entered the endocytic pathway, it must pass through different endosome populations to achieve a successful infection (Figure 8). Endocytic pathway maturation is carefully orchestrated by proteins and lipids that are recruited to the endosomal membrane. Rab GTPase protein family is the major regulator of the endosomal maturation pathway, where each member of the Rab family is specifically located to a different endosomal compartment. Incoming viruses are found in early endosomes (EE) labeled with Rab5 and EEA1 markers from very few minutes after adsorption. In fact, complete encapsidated virions are only found at the level of EE but not in other mature acidic compartments. The inhibition of endosomal acidification with bafilomycin A1 prevents viral decapsidation and only under this condition it is possible to observe complete viruses inside multivesicular endosomes positive for CD63 and late endosomes expressing Rab7. In normal conditions, late endosomes harbor only viral cores lacking the capsid protein.
Viral decapsidation occurs at the acidic intraluminal pH in mature endosomal compartments between 30 and 45 min post infection (mpi). Mature endosomal compartments are multivesicular bodies expressing CD63 that are characterized by the presence of intraluminal vesicles and also, late endosomes expressing Rab7. Dependence on the endosomal maturation for sequential viral uncoating and penetration has been also described for other viruses. Once decapsidated, virus particles expose the inner envelope which allows their interaction and subsequent fusion of this viral membrane with the limiting membrane of the endosomes and naked cores can be released into cytosol in order to start replication. This process is strongly dependent on the cholesterol efflux at the endosomal membrane. In fact, blocking cholesterol transport at this level causes retention of virions inside endosomes, inhibiting infection progression. The inner envelope viral protein pE248R is also involved in viral fusion. This protein shares sequence similarity with some members of the poxviral entry/fusion complex.
Other inhibitors of endosome maturation such as wortmannin, a phosphatidylinositol 3 (PI3)-kinase inhibitor that blocks early endosome fusion, and nocodazole, an inhibitor that disturbs microtubule-dependent endosomal transport also prevent ASF vius infection (Galindo and Alonso, 2017).
5. Epidemiology
5.1 Susceptible hosts
In the natural sylvatic cycle, the soft-bodied, eyeless Ornithodoros ticks (also known as
tampans) are, together with African wild suids, the natural reservoir hosts of ASF virus. They can transmit the virus through their bites.
All members of the pig family (Suidae) are susceptible to infection, but clinical disease
is only seen in domestic and feral pigs, as well as in the closely related European wild boar. Wild African suids are asymptomatic carriers of ASF and act as the reservoir of the virus in parts of Africa. These include warthogs (Phacochoerus africanus and P. aethiopicus), bushpigs (Potamochoerus porcus and Potamochoerus larvatus) and giant forest hogs (Hylochoerus meinertzhageni) (Beltran-Alcrudo et al., 2017).
5.2 Transmission
Sylvatic cycle
This cycle involves the natural hosts of the ASF virus, i.e. warthogs and soft ticks of the Ornithodoros moubata complex, which act as biological vectors in Southern and Eastern Africa. However, information is scarce for other African regions. Also, the precise role of other African wild suids, e.g. bushpigs, still needs to be clarified.
The ASF virus is maintained by tick-to-warthog transmission (Figure 10). Warthogs are
infected by Ornithodoros bites in the first 6-8 weeks of life, while in the burrow.
They subsequently develop sufficient viraemia to infect other ticks. Following a short period when the virus is present in their bloodstream (2-3 weeks), the young warthogs recover, showing no clinical signs. In endemic areas, up to 100 percent of warthogs may have antibodies to ASF virus. Virus can usually be recovered from the lymph nodes of warthogs of any age, although viraemia sufficient to infect ticks has only been found in neonates from burrows. It is likely that warthogs experience repeated infections when ticks feed on them, with low levels of virus remaining latent in the lymph nodes.
Tick populations can remain infected and infective for long periods due to transstadial,
venereal and transovarial transmission of the virus in the tick population, allowing the virus to persist even in the absence of viraemic hosts. Infected ticks play an important role in the long-term maintenance of the disease, surviving for months in burrows and up to several years after feeding on an infected host (Beltran-Alcrudo et al., 2017).
Domestic cycle
In this cycle, the most commonly reported scenario in domestic pigs, the virus is maintained in pigs in the absence of wild suids and ticks. The virus may spread through direct contact via the oro-nasal route after contact with excretions from infected pigs, through ingestion of pork or other contaminated products, or indirectly through fomites. The virus is transmitted from one farm to the next almost exclusively due to human intervention, e.g. movement of animals or equipment, the feeding of infected materials, etc. This transmission route requires the existence of large, continuous populations of pigs for the virus to remain in circulation. However, even in the absence of infected pigs, sometimes the persistence of the virus in refrigerated or frozen meat allows it to persist for long periods of time, and reappear once those meat products are fed as swill (Beltran-Alcrudo et al., 2017).
Wild boar cycle
In Eastern Europe, the Caucasus and Sardinia, wild boar populations play an important role in the maintenance of viral circulation and infection, particularly where there are free-ranging or scavenging populations of pigs in the area, or through some other biosecurity breaches, such as infected feed or leftovers being dumped, fences that allow nose-to-nose contact, etc. Some role may also be played by transportation of wild boar to hunting ranches and/or for management purposes, as well as by hunters.
The exact role of wild boar is, however, still not completely understood. In the Caucasus
and the Russian Federation, where wild boar densities are relatively low, their infection was not sustained for long periods, and mainly stemmed from spillover from domestic pigs.
However, as ASF progressed westward into the dense wild boar populations of Poland
and the Baltic States, sustained transmission and continuous outbreaks were observed throughout the year. In these areas, wild boar are believed to be the true epidemiological reservoir of the virus, with most cases detected in the summer months.
In parts of Eastern Europe, where temperatures remain below 0°C for much of the
winter, a new, previously unseen epidemiological pattern is unfolding. The virus, present
in infected carcasses in fields or forests, remains infective until the spring, when wild boar (and potentially free-ranging pigs, although uncommon) may scavenge on such remains and become infected.
Human interventions, such as hunting, supplementary feeding, fencing, etc., have
profound consequences on how epidemics evolve in wild boar populations. Hunting may
lead to wild boar spreading ASF while escaping to other areas, but it can be also very
useful in regulating the density of animals (and thus virus transmission). Different types of hunting also have different effects, e.g. driven hunts, targeting of females, etc. Similarly, supplementary feeding may increase transmission by encouraging high numbers of wild boar to congregate in feeding areas, while also allowing more wild boar to survive harsh winter conditions (Beltran-Alcrudo et al., 2017).
Tick-pig cycle
In the Iberian Peninsula, ASF virus readily found a suitable host in Ornithodoros erraticus, local ticks that lived in pig shelters. The ticks then became involved in the maintenance of ASF virus and its transmission to pigs, despite the absence of African wild pigs. The cycle has also been described in parts of Africa, where it is well documented in Malawi, Madagascar and Mozambique, although ticks probably do not play a prominent role in virus transmission within pig. Several Ornithodoros tick species have been shown to be competent vectors of ASF virus both in the field and experimentally (Table 1). However, what happens in the laboratory does not necessarily reflect what happens under field conditions. For Ornithodoros ticks to become competent vectors under field conditions, they need pigs as their preferred hosts, failing which natural transmission is likely to remain limited. Vector competence may also vary greatly inside species, or groups of closely related species, according to distinct population features. Although Ornithodoros ticks have been reported in currently infected areas in the Caucasus and southern parts of Eastern Europe, there is no indication of their involvement in the ASF epidemic cycle or of whether they could actually transmit the disease (Beltran-Alcrudo et al., 2017).
Table 1.
Ornithodoros ticks’ geographic distribution and role in the transmission of ASF
(Beltran-Alcrudo et al., 2017)
Ornithodoros | Geographical | Trans-ovarial | Trans-stadial | To | Comments |
O. erraticus | Iberian Peninsula and Northern Africa | No | Yes | Yes | Inhabits pigsties and maintains a cycle in domestic pigs |
O. moubata | Southern and eastern | Yes | Yes | Yes | Depending on the subspecies, it may inhabit warthog burrows and maintain the sylvatic cycle in warthogs, but can also inhabit pigsties (maintaining a cycle in |
O. puertoricensis | Caribbean | Yes | Yes | Yes | Proved an efficient vector, but no virus detected despite large numbers collected in Haiti and Dominican Republic after ASF outbreaks |
O. coriaceus | USA | No | Yes | Yes | Proved an efficient vector |
O. turicata | USA | ? | ? | Yes | Proved able to transmit the virus to pigs experimentally |
O. savignyi | Africa | ? | ? | Yes | Is a desert tick not associated with pigs or warthogs |
O. sonrai | Sahel in North Africa (southward extension of range to south Senegal) |
|
|
| ASF viral genome detected by PCR in four out of 36 ticks on |
ASF transmission and resilience of the ASF virus
The incubation period represents the time from infection (i.e. when the virus enters the
animal) to disease (i.e. when the animal shows clinical signs). For ASF, it is between four
and 19 days, depending on the virus, host and route. Virus excretion can begin up to two
days prior to the appearance of clinical signs. The period when the pig is shedding virus can vary depending on the virulence of the ASF virus strain involved – pigs infected with less virulent ASF virus strains could be persistently infectious for more than 70 days post-infection.
The virus is shed in saliva, tears, nasal secretions, urine, faeces, and secretions from the genital tract. Blood, in particular, contains large amounts of virus. Pigs can therefore become infected by contact with many different infected sources, mainly infected pigs, pork, and other pig-derived products (e.g. swill), and fomites (e.g. bedding). These infected animals and contaminated materials can be transported over long distances by vehicles and people.
Although ASF is associated with high lethality (most animals infected die), it is not as infectious as some other transboundary animal diseases such as foot-and-mouth disease. That means ASF usually spreads slowly within the herd, and some animals may not be affected.
In a suitable, protein-rich environment, the ASF virus is stable over wide ranges of temperatures and pH levels for long periods, as well as resistant to autolysis and various
disinfectants. Thus neither putrefaction, nor the maturing process, nor freezing of meat
inactivates the agent. Consequently, the virus survives in excretions, carcasses, fresh meat,
and certain meat products for varying periods of time. It may remain infective for at least
11 days in faeces, for 15 weeks in chilled meat (and probably longer in frozen meat), and
for months in bone marrow or cured hams and sausages unless they have been cooked or
smoked at high temperature (Table 2). This has very important implications for ASF spread.
Table 2. Resilience of ASF virus across a variety of environmental conditions (Beltran-Alcrudo et al., 2017)
Item | ASF virus survival time |
Meat with and without bone and ground meat | 105 days |
Salted meat | 182 days |
Cooked meat (minimum of 30 minutes at 70oC) | 0 |
Dried meat | 300 days |
Smoked and deboned meat | 30 days |
Frozen meat | 1,000 days |
Chilled meat | 110 days |
Offal | 105 days |
Skin/fat (even dried) | 300 days |
Blood stored at 4oC | 18 months |
Faeces at room temperature | 11 days |
Putrefied blood | 15 weeks |
Contaminated pig pens | 1 month |
Undercooked, insufficiently smoked, dried, and salted pork, as well as blood, carcasses, and carcass meal can be infective if fed to pigs or discarded in communal waste sites where pigs or wild boar may feed. Cooking at 70°C for 30 minutes inactivates the virus.
The introduction of new pigs into a herd or piggery often results in individuals fighting
and biting each other. In the case of free-ranging or scavenging animals, infection can
result from contact with infected roaming pigs, wild boar, their carcasses, or food leftovers. Additionally, using the same needle to vaccinate or treat several pigs can transmit the virus. Transmission via artificial insemination has not been proven, but may take place.
Vector-borne transmission is also possible through bites from infected Ornithodoros
species. Certain blood-sucking insects, namely Stomoxys calcitrans, have been shown to be able to retain and transmit ASF virus for at least 24 hours after feeding on a sick pig, which is particularly relevant for transmission within herds. Infection via large bodies of water such as lakes and rivers is unlikely as the virus rapidly becomes diluted and will not be present at infective levels.
6. Pathogenesis
The sites of primary ASF virus replication are the monocytes and macrophages of the lymph nodes nearest the point of virus entry. In the case of oral exposure, the monocytes and macrophages of the tonsils and mandibular lymph nodes are the first involved. Subsequently the virus spreads via the blood and/or lymphatic system to sites of secondary replication: lymph nodes, bone marrow, spleen, lung, liver, and kidney. Viremia usually begins 4–8 days post-entry and, because of the absence of fully neutralizing antibodies, persists for weeks or months.
While ASF virus replicates mostly in monocytes and macrophages, it also replicates in endothelial cells, hepatocytes, renal tubular epithelial cell, and neutrophils. No infection has been observed in T or B lymphocytes. The virus particle enters susceptible cells by receptor‐mediated endocytosis and replicates in distinct areas of the cytoplasm close to the nucleus. Studies on the interaction of ASF virus with monocytes and derived macrophage subsets showed that virulent isolates have evolved mechanisms to counteract activated macrophage responses, thereby promoting viral survival, dissemination in the host, and ASF pathogenesis.
Most ASF virus isolates bind to red blood cell membranes and platelets and cause hemadsorption in affected pigs. Some isolates, however, do not induce hemadsorption.
The hemorrhaging observed in the final stages of the ASF acute form is believed to reflect phagocytosis of the endothelial cells in which the virus is replicating. In contrast, hemorrhaging in ASF subacute form reflects mainly an increase in vascular permeability. Lymphopenia in ASF acute form is associated with lymphocyte apoptosis, mainly in the T area of lymphoid organs. This apoptosis does not appear to involve viral replication, since no evidence of replication in T or B cells has been reported; instead, other processes are likely to be involved, perhaps triggered by cytokines or apoptotic mediators released by ASF virus infected macrophages.
ASF subacute form is characterized by transitory thrombocytopenia. The alveolar edema observed in the final stages of acute and subacute forms of ASF is the primary cause of ASF related death. This edema results from the activation of pulmonary intravascular macrophages (Sánchez‐Vizcaíno et al., 2019).
6.1 ASF virus cellular tropism
ASF virus has a restricted cellular tropism. The primary target cells for replication are macrophages and monocytes, although replication in dendritic cells has also been reported. These cell types play a key role in induction of innate immune responses, responding to specific pathogen associated signals. These cells also present antigen to T cells in order to activate the adaptive immune response, which is vital for development of a protective immune response. Macrophages are specialised in recognition and destruction of pathogens and initiation of an inflammatory response. Dendritic cells respond to inflammatory responses to become efficient antigen presenting cells and migrate to T cell areas in secondary lymphoid tissues.
Replication in macrophages and monocytes
Cells typical of monocytes and macrophages, at an intermediate to late stage of differentiation, are the main cells infected both in vitro and in vivo in pigs. The virus enters these cells by either receptor-mediated endocytosis, into clathrin-coated pits, or by macropinocytosis, a less specific mechanism. The restricted cellular tropism suggests that receptor-mediated endocytosis is the main mechanism of entry, although the cellular receptor(s) for binding and entry are unknown. Earlier reports suggested that CD163 may be a receptor for ASF virus but results showed that deleting the CD163 gene from the pig genome did not restrict virus replication in macrophage cultures and did not result in reduced virulence in pigs. The complex ASF virion multi-layered structure adds further complexity to these questions. Both the intracellular mature and the extracellular enveloped forms of the virus are infectious. The outer envelope, which is gained as the virus buds through the plasma membrane, is lost when the virus particles move to the acidic environment of late endosomes. The inner virus envelope fuses with the endosomal membrane releasing the virus core particle into the cytoplasm to initiate the replication cycle. Several virus proteins have been identified that are important in the binding and entry process including p54/pE183 L, p30/pCP204 L and p12/pO61R but the cellular receptors are not known.
Replication in dendritic cells
Porcine dendritic cells can be split into two main populations: conventional DCs (cDCs) and plasmacytoid DCs (pDCs). It is cDCs that are classified as professional antigen presenting cells. The pDCs are specialist type I IFN producing cells, which is key in the maturation of DCs through upregulating MHC class I and II expression and initiation on the adaptive immune response.
There is some evidence that dendritic cells are susceptible to ASF virus infection. Initially it was shown that skin-derived DCs were susceptible in vitro, followed by the identification of ASF virus antigens in interdigitating DCs (iDCs) in the mandibular lymph nodes at 3 days post-infection. More recently it has been shown that monocyte derived dendritic cells (MoDCs) are susceptible to infection with both virulent and attenuated strains of ASF virus. However, upon maturation with IFNα, there is a decreased susceptibility to infection with attenuated strains. In contrast to this, maturation with TNFα led to an increased susceptibility to infection with virulent isolates. It was also indicated that ASF virus infected pDCs could be a source of type I interferon in in vitro infections. This is a potential explanation of the source of high levels of type I interferon in the serum during acute ASF virus infections. The impact of ASF virus infection on dendritic cell function has been little studied (Dixon et al., 2019a).
6.2 Macrophage responses to ASF virus
Macrophages have extraordinary plasticity and can adopt different phenotypes and functions in response to intercellular signals. This cascade of new adaptation includes activation of phagocytosis, increased cell size and subsequently activation of different secretory signals, including cytokines and chemokines.
Overcoming barriers to replication in the monocyte/macrophage
To replicate in the hostile, highly-oxidising, environment of the macrophage cytoplasm, ASF virus codes for enzymes involved in a DNA
base excision repair (BER) pathway. DNA damage can result in introduction of potentially lethal mutations in the virus genome or, inhibit activity of the virus DNA or RNA polymerases to reduce virus replication. Components of this BER pathway, including the repair DNA polymerase X, AP endonuclease
and DNA ligase have been shown to be required for replication in macrophages, but not in tissue culture cells, highlighting the critical role of the BER pathway in macrophages (Table 3). Components of the BER pathway are packaged in virus particles, ready for use early during replication when the virus core particles enter cells.
Table 3. Non-essential genes identified on the ASF virus genome (Dixon et al., 2019a)
Gene deleted | Function | Isolate | Growth in cells | Virulence in pigs |
DNA repair pathway, genome integrity and nucleotide metabolism | ||||
Q174L | DNA repair | BA71 V (a) | Required for efficient macrophage growth | ND |
E296R | AP endonuclease | BA71 V (a) | Required for macrophage growth | ND |
E165R | dUTPase | BA71 V (a) | Required for macrophage growth | ND |
A240L | Thymidine kinase | Malawi (v) | Required for macrophage growth | Attenuated |
Type I interferon response | ||||
MGF360 | Type I Interferon | Benin 97/1(v) | No effect | Attenuated |
MGF360 | Type I | Pr4 (v) | No effect | Attenuated |
MGF360 | Type I | Georgia (v) | No effect | Attenuated |
DP96R (UK) | IFN inhibitor | Malawi (v) | No effect | Attenuated |
EP402R/CD2v | Binding to red blood cells | BA71 (v) | No effect | Attenuated |
EP402R/CD2v/8-DR | Binding to red blood cells | Malawi LIL20/1(v) | No effect | Delay in clinical signs |
B119L (9 G L) | Morphogenesis | Malawi (v) | Reduced replication | Attenuated |
B119L (9 G L) | Morphogenesis | Georgia (v) | Reduced replication | At low doses attenuated and Induced protection |
DP96R (UK) | Georgia (v) | Reduced replication | Attenuated | |
MGF 360 | IFN inhibitor | Georgia (v) | Reduced replication | Attenuated |
A224 L/4CL | IAP apoptosis inhibitor | Malawi (v) | No effect | No reduction in virulence |
DP71 L/NL | Malawi (v) | |||
DP71 L/NL | E70 (v) | Attenuated | ||
L83L | IL-1beta binding protein | Georgia (v) | No effect | No reduction in virulence |
DP148R | Benin 97/1 (v) | No effect | Attenuated | |
EP153R | C-type lectin | Malawi (v) | No effect | No reduction in virulence |
A238L | Inhibitor of inflammatory responses | Malawi (v) | No effect | No reduction in virulence |
L11L | Transmembrane | Malawi (v) | No effect | No reduction in virulence |
The virus encoded nucleotide metabolism enzyme, thymidine kinase, is also important for efficient replication in macrophages, but not in tissue culture cells. This is presumably to increase pools of dNTPs that are required for virus genome replication, but present at relatively low levels in non-dividing macrophages. Deletion of this gene from the virulent Georgia isolate attenuated the virus in pigs, most likely due to the restricted ability of the virus to replicate in macrophages (Table 3) (Dixon et al., 2019a).
6.3 Pathogenesis in domestic and wild suids
The host range of ASF virus is restricted to suids and soft ticks of the Ornithodoros species. In its wild suid hosts in Africa, ASF virus infection causes mild clinical signs and can result in longer-term persistent infections. In contrast, most ASF virus isolates cause an acute hemorrhagic fever, with a case fatality rate approaching 100%, in domestic pigs and wild boar. Diseases observed in domestic pigs and wild boar include acute and peracute forms, which are caused by highly virulent isolates and result in death within 4 to 15 days postinfection. Moderately virulent isolates cause lower case fatality (30–70%). Low-virulence isolates result in low or no case fatalities and absence of vascular lesions. However, signs of chronic disease can be observed. The clinical signs of acute ASF include high fever, loss of appetite, and increasing lethargy and morbidity. Bloody diarrhea, vomiting, and abortion may also be observed. Infection is associated with very high levels of virus in blood (up to 109 TCID50/mL) and tissues. Wild boars (Sus scrofa) show the same acute signs of disease as domestic pigs. Most isolates circulating in Europe, the Russian Federation, and Asia cause the acute form of disease, although some reduced-virulence isolates have been obtained from infected wild boar in the Baltic States. Animals that recover from disease may remain infected for several months (Dixon et al., 2020).
7. Clinical signs and pathology
Wild African pigs are extremely resistant to clinical disease and do not generally present lesions. Domestic pigs and European wild boars exhibit a wide range of clinical signs from acute to chronic. ASF may resemble several other pig diseases, especially classical swine fever (hog cholera) and erysipelas.
The incubation period ranges from 4 to 19 days in natural infections but only 2–5 days in experimental infections, depending on the virus dose and inoculation route. Clinical manifestations of ASF depend on the virulence of the isolate, exposure dose, and route of infection. Highly virulent ASF virus isolates are mainly involved in the peracute and acute forms of the disease. Moderately virulent isolates can generate a wide range of clinical forms: acute, subacute, and chronic or inapparent. Low virulent isolates may induce subacute, chronic, or inapparent disease.
Morbidity ranges from 40 to 85%, depending on whether the virus isolate causes acute or subacute disease, the virulence of the isolate, the route of exposure, and the presence or absence of bleeding (epistasis or hemorrhagic diarrhea). Highly virulent isolates may cause 90–100% mortality, moderately virulent isolates cause 20–40% mortality in adult animals and 70–80% in young animals, and lowly virulent isolates produce 10–30% mortality (Sánchez‐Vizcaíno et al., 2019).
Peracute ASF
Highly virulent strains are typically responsible for this clinical course, characterized by a very rapid clinical course, with high fever (up to 42oC), anorexia, lethargy, and sometimes sudden death without signs of disease. This is often observed when the virus enters a naïve farm causing death of some animals before the explosion of clinical cases. Some animals can show respiratory distress due to the high fever, but no gross lesions are usually found at the post mortem examination (Salguero, 2020).
Acute ASF
This clinical form is cause by highly or moderately virulent isolates, and it is the typical course observed in naïve farms very quickly after the first fatal cases are reported. The clinical course is characterized by high fever, with temperatures of 40–42oC, lethargy, anorexia, and inactivity (Figure 13A). The affected animals tend to bunch up together. Many affected animals show a centripetal cyanosis, easily found in the ears (Figure 13B), snout (Figure 13C), limbs (Figure 13D), abdomen, tail, and perianal area. Respiratory distress is usually observed, with severe pulmonary oedema in animals affected by highly pathogenic isolates. Skin lesions are frequent, with presence of petechial hemorrhages or ecchymosis (Figure 13E). Other clinical signs may include nasal discharges, sometimes stained with blood (epistaxis), vomiting, and diarrhea, that can be also blood-stained (melaena), causing black-colored stains in the perianal area of the animal (Figure 13F). Abortions may occur in pregnant sows and the mortality rates may reach up to 100% in affected farms within 7 days of the onset of the disease (Salguero, 2020).
associated to very high hyperthermia (41–42◦C). (C) Cyanosis in the snout and lips in acute ASF. (D) Cyanosis in the limbs in acute ASF. (E) Multifocal petechiae and
ecchymosis in the skin in acute ASF. (F) Blood-stained perianal area in a pig affected by subacute ASF. (G) Severe hydropericardium (arrow) in subacute ASF. (H) Moderate to severe ascites (arrow) in subacute ASF (Salguero, 2020).
At the post mortem examination, the most characteristic lesion of acute ASF is the hemorrhagic splenomegaly, with a very enlarged spleen, dark in color and friable at sectioning, occupying a large space within the abdominal cavity (Figures 14A, B). The second most important lesion described in acute ASF is a multifocal hemorrhagic lymphadenitis. Lymph nodes can have multifocal or extensive hemorrhages that can produce a marbled appearance (Figure 14D). The most affected lymph nodes are the gastrohepatic (Figure 14E), renal (Figure 14F), and other abdominal lymph nodes as ileocaecal (Figure 14G), and mesenteric (Figure 14H). Hemorrhages may also be observed with less frequency in other lymph nodes, such as submandibular, retropharyngeal, or inguinal.
Petechial hemorrhages are often observed in the kidney surface (Figure 15A) and at sectioning. Other lesions can also be observed, mostly hemorrhages in the mucosa or the serosa of other organs, as the large (Figure 15E) and small intestine (Figure 15F), the epicardium in the heart (Figure 15G), or the urinary bladder (Figure 15H) (Salguero, 2020).
Very large, dark colored spleen with rounded edges (hemorrhagic splenomegaly), and occupying a large volume of the abdominal cavity in acute ASF. (C) Multiple
areas of partial hemorrhagic splenomegaly in the spleen from an animal with subacute ASF. (D) Multifocal hemorrhages in a lymph node with a marbled appearance in acute ASF. (E) Severe hemorrhagic lymphadenopathy in the gastrohepatic lymph node (arrow) in acute ASF. (F) Severe hemorrhagic lymphadenopathy in the renal lymph node (arrow) in acute ASF. (G) Severe hemorrhagic lymphadenopathy in the ileocaecal lymph node (arrow) in acute ASF. (H) Moderate hemorrhagic lymphadenopathy in the mesenteric lymph node (arrow) in acute ASF (Salguero, 2020).
Multifocal areas of lung consolidation and pulmonary oedema in subacute ASF. (D) Multifocal pneumonia with dark color areas in the diaphragmatic lobe of the lung in
subacute ASF. (E) Severe extensive hemorrhagic colitis in subacute ASF. (F) Multiple petechial hemorrhages in the serosa of the small intestine in acute ASF. (G)
Multiple petechial ad ecchymotic hemorrhages in the epicardium (arrowhead) together with severe hydropericardium (arrow) in subacute ASF. (H) Multiple petechial
hemorrhages in the mucosa of the urinary bladder in acute ASF (Salguero, 2020)
Subacute ASF
This clinical form is usually observed in animals infected by moderately virulent isolates, with similar clinical signs as those observed in acute ASF, although normally less marked. Affected pigs show moderate to high fever and the mortality rate ranges from 30 to 70%, with pigs dying at 7–20 after infection. The vascular changes, mostly hemorrhages and oedema, in the subacute form of the disease can be more intense than the acute form. The death of affected animals may happen at two different stages: (a) during an initial thrombocytopenia and leukopenia, or (b) during a “recovery” phase, observed in young animals, causing erythrodiapedesis induced by vasodilation.
At the post mortem examination, animals show hydropericardium (Figure 13G), ascites (Figure 13H), and multifocal oedema, very characteristic in the wall of the gall bladder or in the perirenal fat (Figure 16B). Some animals may show hemorrhagic splenomegaly as described for the acute form of the disease, but many animals will show partial splenomegaly, with patches of spleen affected and other areas unaffected (Figure 14C). A multifocal hemorrhagic lymphadenitis can also be observed with multiple lymph nodes in all areas of the body showing the hemorrhages and the “marble” appearance. Petechial hemorrhages can also be observed in the kidney. Multifocal pneumonia is also observed with patches of consolidation and dark color in the lung (Figures 14 C, D). This lesion can also be attributed to secondary infections due to the state of immunosuppression induce by ASF virus (Salguero, 2020).
Chronic ASF
This clinical form is caused by the infection of low virulence isolates and has been observed, quite infrequently, in the Iberian Peninsula and the Dominican Republic. It has been hypothesized that this low virulence isolates, and the associated chronic form, has evolved from ASF virus isolates employed in early vaccine trials carried out in the Iberian Peninsula in the 1960’s. The evolution of highly and moderately virulent isolates in other areas where the virus has been present for long periods of time has not produced this chronic form of the disease. This clinical form is characterized by multifocal necrosis in the skin and arthritis, growth retardation emaciation, respiratory distress and abortion. No vascular changes are observed in the chronic form of ASF, and many observed lesions are associated with bacterial secondary infections, inducing fibrinous polyserositis, necrotic, or chronic pneumonia, necrosis of the skin, tongue, and tonsils after the bite of an infected soft tick. The virus replicates initially in the tonsils or regional lymph nodes, spreading through the lymph and blood to secondary organs of replication within 2–3 days, and then spreading to the rest of the organs, where virus can replicate in a variety of cells. Monocytes and macrophages are the main target cell for ASF virus. ASF virus is a DNA virus, but the replication occurs within the cytoplasm and not in the nucleus. The infected monocyte-macrophage appears swollen, with margination of the nuclear chromatin (Figures 16 A, B) and showing an intracytoplasmic juxtanuclear inclusion body, identifiable by its pale color when semithin (1-micron) sections are stained with toluidine blue dye (Figure 16 A). These inclusion bodies show viral factories when studied under transmission electron microscopy (Figure 16B). The virus replication induce necrosis in the infected cells and virions are released by budding, and can be observed free in the blood, lymph, and the interstitial tissue. The destruction of monocytes-macrophages in ASF has been attributed to apoptosis or necrosis due to the action of ASF virus. ASF virus genome contain genes involved un programmed cell death both in an inhibitory or an inducing manner. Some of these genes may promote the survival of the infected cells, and apoptosis has been described as the less likely cause of cell death in the infected monocytemacrophage population. ASF is characterized by a massive destruction of the lymphoid organs and tissues, including spleen, lymph nodes, thymus, and tonsils. There is a large proportion of B and T lymphocytes and macrophages undergoing cell death in acute ASF virus infection. The virus replication in the monocyte-macrophages induces an activation in this cell population and an increase in the secretion of proinflammatory cytokines have been observed at the early stages of the disease. The upregulation in the expression of proinflammatory cytokines, including IL-1, TNF-α, and IL-6, and described as a “cytokine storm”, is the responsible mechanism for the massive induction of apoptosis in lymphocytes (Figure 16C) neighboring the activated/infected monocyte-macrophages in tissues. (Salguero, 2020).
8. Diagnosis
8.1 Differential diagnosis
African swine fever does not always manifest itself with the entire set of clinical signs
described in the previous section. Clinical diagnosis can be difficult during the early stages of the disease, or when small numbers of animals are affected. Diagnosing ASF is often speculative, for symptoms may be confused with those of other diseases and/or conditions. Moreover, a number of pig (and wild boar) diseases can cause mortality at the rate observed in an acute ASF outbreak. No diagnosis is conclusive until confirmed by the laboratory. In addition to the top differential diagnoses covered in this chapter, additional conditions to consider may include other generalized septicaemia or haemorrhagic (bruising) conditions.
Classical swine fever (CSF)
The most important differential diagnosis of ASF is CSF, also known as
hog cholera, which is caused by a Pestivirus in the Flaviviridae family. As with ASF, there are various clinical presentations or forms. Acute CSF presents almost identical clinical signs and postmortem lesions to acute ASF, and is also characterized by high fatality rates. Clinical signs may include high fever, lack of appetite, depression, haemorrhages (in the skin, kidneys, tonsils and gall bladder), conjunctivitis, respiratory signs, weakness, huddling, purple discolouration of skin, and death within 2-10 days. The only way to distinguish reliably between them is through laboratory confirmation. It is unwise to attempt vaccination against CSF until the diagnosis is confirmed, as ASF can easily be spread by untrained personnel during a vaccination campaign.
Porcine reproductive and respiratory syndrome (PRRS)
Also known as blue ear disease, PRRS is characterized by pneumonia in growing and finishing pigs and by abortions in pregnant sows. It is often accompanied by fever, skin flushing and in particular by bluish discolouration of the ears. Diarrhoea has also been described. Although mortality due to PRRS is generally not high, highly pathogenic PRRS viruses have decimated pig herds in China, Viet Nam and Eastern Europe over the last few years, associated with high mortality, high fever, lethargy, anorexia, cough, dyspnoea, lameness, and cyanosis/bluing (in ears, limbs and perineum). Necropsy findings include lesions in lungs (interstitial pneumonia) and lymphoid organs (atrophy of the thymus and swelling and haemorrhages in lymph nodes) and petechial haemorrhages in the kidneys.
Porcine dermatitis and nephropathy syndrome (PDNS)
One of the porcine circovirus-2 associated diseases (PCVAD), PDNS usually affects
growers and finishers. Although the clinical signs are strongly suggestive, there is no
specific diagnostic test. The syndrome is characterized by the presence of dark-red to
purplish skin lesions that are often most prominent on the hindquarters and perineal area, although in severe cases the flanks may also be affected. The lesions in the blood
vessel walls are caused by necrotizing vasculitis (inflamed blood vessels), and are easily
distinguished microscopically from those of ASF. The disease is also accompanied by
anorexia, depression and severe nephrosis (inflamed kidney), which is usually the cause
of death. Lymph nodes may also be enlarged. Morbidity is generally low but affected
pigs very often die.
Erysipelas
This bacterial disease caused by Erysipelothrix rhusiopathiae affects pigs of all ages and is as likely to affect pigs in small-scale and extensive farms as in commercial, intensive units. It can manifest itself in either acute or subacute forms. The acute form, usually seen in younger pigs, is characterized by sudden death, although mortality is usually much lower than in ASF. Two or three days after infection, affected pigs may show very characteristic diamond-shaped skin lesions associated with necrotizing vasculitis (inflamed blood vessels). In adult pigs this is usually the only clinical manifestation of the disease. As with acute ASF, the spleen may be congested and markedly enlarged. Other necropsy findings include congestion in lungs and peripheral lymph nodes, as well as haemorrhages in the cortex of the kidneys, heart and serosa of the stomach. Bacterial isolation can confirm the diagnosis and pigs respond well to treatment with penicillin. The microscopic changes differ from those typical of ASF.
Aujeszky’s Disease
Aujeszky’s disease, also known as pseudorabies, causes reproductive and severe neurological issues in affected animals, often leading to death. Although nearly all mammals can be infected, pigs are most frequently affected and are the reservoir host. Younger animals are the most severely affected, with mortality rates reaching 100 percent during the first two weeks of age. Piglets usually have a fever, stop eating, and show neurological signs (trembling, seizures, paralysis), and often die within 24-36 hours. Older pigs (over two months) may show similar symptoms, but usually have respiratory signs and vomiting, and are less likely to die. Sows and boars primarily develop respiratory signs, but pregnant sows can abort or give birth to weak, trembling piglets. Focal necrotic and encephalomyelitis lesions occur in the cerebrum, cerebellum, adrenals and other viscera such as lungs, liver or spleen. In fetuses or very young piglets, white spots on the liver are highly characteristic of infection by the virus.
Salmonellosis (and other bacterial septicaemias)
Younger pigs are usually affected. Animals treated in time may respond to antimicrobial
therapy. Confirmation of the diagnosis is by bacterial culture. Features in common with ASF include fever, loss of appetite, respiratory or gastrointestinal disorders, and a congested, fevered carcass at slaughter. Animals may die 3-4 days post-infection. Pigs dying from septicaemic salmonellosis show cyanosis of the ears, feet, tail and abdomen. Necropsy findings may include petechial haemorrhages in the kidneys and on the heart’s surface, enlarged spleen (but with normal colour), swelling of mesenteric lymph nodes, enlargement of the liver, and congestion of the lungs.
Poisoning
When a large number of pigs die suddenly, the possibility of poisoning should be considered. Few poisons result in the severe bleeding seen in ASF. Although coumarin-based rat poisons such as warfarin can cause widespread bleeding, they are unlikely to affect more than a few pigs in the herd. Certain fungal toxins found in mouldy feed such as aflatoxin and Stachybotrys toxin may cause haemorrhage and severe mortality. Accidental or malicious poisoning with pesticides can result in the death of pigs of all ages, but the death of all pigs in the space of 24-48 hours, usually with few if any clinical signs or postmortem lesions, should serve to distinguish such events from ASF. Poisoning is unlikely to be accompanied by fever (Beltran-Alcrudo et al., 2017).
8.2 Laboratory diagnosis
Detection of ASF virus
ASF virus genome detection by polymerase chain reaction (PCR)
Polymerase chain reaction (PCR) is used to detect the ASF virus genome in porcine samples (blood, organs, etc.) and ticks. Small fragments of viral DNA are amplified by PCR to detectable quantities. All validated PCR tests allow viral DNA detection even before the appearance of clinical signs. PCR enables the diagnosis of ASF to be made within hours of sample arrival to the laboratory. PCR provides a sensitive, specific, and rapid alternative to virus isolation for the detection of ASF virus. PCR provides higher sensitivity and specificity than alternative methods for antigen detection, such as the antigen enzyme-linked immunosorbent assay (ELISA) and the direct fluorescent antibody test (FAT). However, the extreme sensitivity of the PCR makes it susceptible to cross contamination, and proper precautionary measures should be taken to minimize and control this risk. Conventional and real-time PCRs recommended by the OIE in the Manual of Diagnostic Tests and Vaccines for Terrestrial Animals (2016) have been fully validated over time and are useful tools for routine diagnosis of the disease. Other real-time PCR procedures have proved to provide higher sensitivity than OIE-prescribed, real time PCR methods for ASF virus genome detection in recovered animals. Primer sets and probes used in these molecular techniques are repeatedly designed within the VP72 coding region, a well-characterized and highly conserved region of the ASF virus genome. A wide range of isolates belonging to all the 22 known p72 virus genotypes can be detected with these PCR assays, even in inactivated or degraded samples. PCR is the tool of choice in the case of peracute, acute, or subacute ASF infections. Furthermore, since PCR detects the viral genome, it may be positive even when no infectious virus is detected by virus isolation, making it a very useful tool for the detection of ASF virus DNA in pigs infected with low- or moderately virulent strains. Although PCR is not informative about the infectivity of the virus, it can provide quantitative information (Beltran-Alcrudo et al., 2017).
ASF virus isolation
Virus isolation is based on the inoculation of sample material onto susceptible primary cell cultures of porcine origin, monocytes, and macrophages. If the ASF virus is present in the sample, it will replicate in the susceptible cells, producing cytopathic effect (CPE) in the infected cells. Cell lysis and CPE usually occur after 48-72 hours of haemadsorption. The importance of this finding relies on its specificity because none of the other pig viruses are capable of haemadsorbing in leukocyte cultures. When the virus replicates in these cultures, most of the ASF virus strains produce the haemadsorption reaction (HAD) due to adsorption of pig red blood cells on ASF virus-infected leukocytes forming “rosettes”.
However, it is important to point out that the CPE, in absence of haemadsorption, could be due to the cytotoxicity of the inoculum, the presence of other viruses such as Aujeszky’s disease virus, or to a non-haemadsorbing ASF virus isolate. In these cases, the presence of ASF virus on the cell sediment must be confirmed by other virological assays such as FAT or by the use of PCR. If no change is observed, or if the results of the FAT and PCR are negative, the supernatant must be sub-inoculated into fresh cultures for up to 3-5 passages before discounting the presence of ASF virus. Virus isolation and identification by HAD are recommended as a reference test for the confirmation of positive results of a prior antigen positive test (ELISA, PCR or FAT). They are also recommended when ASF has already been confirmed by other methods, particularly in the case of a first outbreak of ASF in an area. In addition, virus isolation is essential if the objective is to obtain virus stocks for future molecular and biological characterization studies (Beltran-Alcrudo et al., 2017).
ASF antigen detection by direct fluorescent antibody test (FAT)
The FAT can be used to detect ASF virus antigen in pig tissues. The principle of the test is the microscopic detection of viral antigens on impression smears or thin cryosections of organ material. Intracellular antigens are detected using fluorescein isothiocyanate (FITC)-conjugated specific antibodies. FAT can also be used to detect ASF virus antigen in leucocyte cultures in which no HAD is observed, and can thus identify non-haemadsorbing strains of ASF virus. It also distinguishes between the CPE produced by ASF virus and that produced by other viruses, or due to the cytotoxicity of the inoculum. Positive and negative controls are used to ensure that the slides are interpreted correctly. This is a highly sensitive test for cases of peracute and acute ASF and can be carried out fairly rapidly. It is a robust test, but has been largely replaced by PCR and reagents are no longer widely available. However, it is important to note that in subacute and chronic disease, the FAT has a significantly decreased sensitivity (40%) (Beltran-Alcrudo et al., 2017).
ASF antigen detection by antigen ELISA test
Viral antigens can also be detected using ELISA, which is cheaper to set up than PCR methods and allows large-scale testing of samples in a short time without special laboratory equipment. However, as in the case of the FAT, in subacute and chronic disease the antigen ELISA has a significantly decreased sensitivity. In addition, field samples are often in poor condition and therefore also decrease the sensitivity of the test. It is thus recommended to use the antigen ELISA (or any other ELISA) only as a “herd” test and in conjunction with other virological and serological tests (Beltran-Alcrudo et al., 2017).
Detection of ASF antibodies
Serological assays are the most commonly used diagnostic tests due to their simplicity, comparatively low cost, and their necessitating few specialized pieces of apparatus or facilities. Since there is no vaccine against ASF, the presence of ASF virus antibodies always indicates current or historic infection. Furthermore, ASF virus antibodies appear early after infection and persist up to several years. However, in peracute and acute infections the pig often dies before antibodies become detectable. It is therefore recommended that in the early stages of an outbreak, samples are taken for detection of viral DNA as well. For the detection of ASF antibodies, the recommended tests include the ELISA test for antibody screening followed by the immunoblotting test or indirect fluorescent antibody (IFA) test as confirmation. The antibody detection by indirect immunoperoxidase test can be used as an alternative confirmatory test for the detection of ASF antibodies in porcine sera and in tissue exudate. It can be easily applied to a large number of samples, does not require expensive fluorescence microscope equipment, and provides appropriate sensitivity (Beltran-Alcrudo et al., 2017).
ASF antibody detection by ELISA test
The ELISA test is a very useful technique, widely used for large-scale serological studies of many animal diseases. Some of the most notable characteristics of this method are high sensitivity and specificity indexes, high speed, low cost and easy interpretation of results.
Large populations can be rapidly screened thanks to the automatic equipment available.
The ELISA uses tagging to identify ASF antibodies in serum samples. In this technique,
the antibodies are tagged with certain enzymes. When an antigen and antibody bind to
each other, the enzyme causes a reaction that produces a colour change, thereby identifying the presence of ASF. A variety of commercial and “in-house” methods such as indirect or blocking ELISA tests are currently available for ASF antibody detection. Sera incorrectly handled or badly preserved (due to inadequate storage or transportation) and haemolyzed samples may yield up to 20% false-positive results. Therefore, all positive and doubtful samples by ELISA must be confirmed by alternative serological confirmatory tests. The IB technique is a rapid and sensitive assay for the detection and characterization of proteins. It works by exploiting the specificity inherent in antigen-antibody recognition. This test involves the production of antigenic strips bearing the virus antigens. It involves solubilization, electrophoretic separation, and transferring of proteins onto membranes (usually nitrocellulose). The membrane is overlaid with a primary antibody for a specific target and then with a secondary antibody labelled to visualize the positive reaction.
The first viral proteins that induce ASF-specific antibodies in pigs invariably react by immuno blotting in all the infected animals. Positive reactions begin with sera obtained from animals 7-9 days post-infection, and up to several months post-infection in surviving animals. Sera from animals vaccinated against other viruses can induce false-positive reactions. In those cases, alternative confirmatory tests such as IPT or FAT should be used (Beltran-Alcrudo et al., 2017).
ASF antibody detection by indirect fluorescent antibody (IFA) test
The test is based on the detection of ASF antibodies that bind to a monolayer of green
monkey kidney cells infected with an adapted ASF virus. The antibody-antigen reaction is detected by a labelled fluorescein conjugate. Positive samples show specific fluorescence in the cytoplasm of the infected cells. The IFA is a rapid technique with high sensitivity and specificity for the detection of ASF antibodies from sera, plasma or tissue exudates (Beltran-Alcrudo et al., 2017).
ASF antibody detection by indirect immunoperoxidase test (IPT)
The IPT is an immune-cytochemistry technique on fixed cells to determine the antibody antigen complex formation through the action of peroxidase. In this procedure, green monkey kidney cells are infected with ASF virus isolates adapted to these cell cultures. The infected cells are fixed and used as antigens to determine the presence of the specific antibodies against ASF in the samples. As is the case with FAT, IPT is a rapid technique with high sensitivity and specificity for the detection of ASF antibodies from sera, plasma or tissue exudates. Interpretation of the results is easier than FAT, because of the enzymatic visualization system employed. In conclusion, current available diagnostic tests allow one to confidently diagnose ASF by combining both virus and antibody detection. Real-time PCR is the most widely used for virological diagnosis, providing sensitive, specific, and swift detection of ASF virus DNA. Due to the possibility of a cross-contamination, a unique positive PCR result from a single animal in a free area (e.g. a wild boar), or a single positive PCR result within a group of animals, should be confirmed by additional virus detection tests and should be combined with serological, pathological and epidemiological findings. Since PCR detects viral DNA presence and not live virus, it is highly recommended to get virus isolation from infected samples prior to the confirmation of an outbreak if a new region is affected. Keeping test limitations in mind, validated ELISA tests are the technique of choice for ASF antibody detection, particularly for screening serum samples. Confirmatory tests such as IB, IFA or IPT are crucial to identify false-positive ELISA results. In addition, IFA and IPT are the recommended techniques for the analysis of tissue exudates and plasma samples, providing a complete picture of the epidemiology, and allowing to determine the time of infection. An accurate ASF diagnosis must include the virological and serological results together with the clinical, pathological, and epidemiological findings. Summarizes the characteristics of the main laboratory diagnostic techniques for ASF (Beltran-Alcrudo et al., 2017).
9. Treatment
There is no treatment. All infected animals must be isolated and culled immediately upon confirmation of presence of the virus.
10. Control and prevention of ASF
10.1 Control and prevention
To prevent the introduction of ASF, movement restrictions regarding pigs, pork, blood and other products from pigs kept in affected areas as well as potentially contaminated material, vehicles etc. are in place. Following European Commission, necessary biosecurity measures are defined, e.g. swill feeding, in commercial pig farms as well as in wild boars, must be prohibited, especially in high risk areas. Direct or indirect contact to wild boar or to any by products has to be avoided. The measures that have to be taken in a case of ASF suspicion or an actual outbreak in the European Union have been specified by European Commission.
When an outbreak of ASF in a farm has been confirmed, all pigs of the premise must be culled. In addition, further measures like the safe disposal of all potentially contaminated material, restriction (minimum radius of 3 km) and surveillance (minimum radius of 10 km) zones with movement restrictions for pigs and products of porcine origin have to be set up. Specific regulations have been defined for both zones in European Commission (Schulz et al., 2017).
10.2 Cleaning and disinfection procedures
Cleaning and disinfection (C&D) procedures are fundamental for pathogen inactivation, to prevent the spread of the disease and to facilitate the repopulation after an outbreak. Cleaning represents one of the most important steps in the C&D process. It removes over 90% of microorganisms when properly performed and improves the disinfection efficacy. The C&D protocol described below follows a general scheme to apply in a systematic manner (FAD PReP, 2018) also within the framework of an ASF control program. EU Council Directive 2002/60/EC (EC, 2002) establishes that in case of ASF, the destruction of carcasses shall be followed by the thorough C&D of all premises, vehicles and equipment. These operations shall be conducted under the supervision of the Veterinary Authority and C&D must be documented in the holding or vehicle register and where official approval is required, be certified by the supervising official veterinarian. Adequate safety measures must be taken during the implementation of the disinfection processes and all team members equipped with appropriate protective clothing, boots, hats, visors, gloves and respirators (FAD PreP, 2014).
10.2.1 Cleaning
Cleaning protocol comprises the following phases:
- Dry cleaning
- Pre-soaking
- Wet cleaning
- Rinsing and drying
10.2.2 Disinfection
In areas affected or at risk of introducing ASF the disinfectants to choose for the control of the disease must be effective against the ASFV and approved by the official veterinarian. In case of ASF outbreak the procedure to follow are described in CD 2002/60/EC
- Application
According to Van Immerseel et al. (2018) two main ways of disinfectant application can be distinguished: wet (surface) and dry disinfection. Surface disinfection is often carried out with high pressure. In the case of dry disinfection (thermal fogging), a highly concentrated disinfectant is heated and subsequently converted to a fog by a fogger. Disinfection applied in a fumigation stage may ensure elimination of pathogens in difficult-to-reach zones and can be performed where it is possible to seal the building completely. The amount of disinfectant necessary varies considerably, it is considered that for a polished, non-porous floor, 100 ml/m3 of disinfectant/chemical is sufficient (FAO, 2001). It is very important to calculate in the right way the amount of solution needed to disinfect an animal premise (Van Immerseel et al., 2018).
- Contact time
Adequate contact time must be allowed for the process to be effective and it can vary according to the surface to be treated (FAD PReP, 2014; FAD PReP, 2018). In some cases, the disinfectant may need to be applied again to keep the surface wet for the required contact time and it must remain on the surfaces for at least the time indicated in the instructions (OIE, 1995). In case of ASF, disinfectants must remain on the surfaces for at least 24 hours (EC, 2002).
- Drying
Cleaned and disinfected premises should also have a period of down time following the disinfection procedures. Premises should remain empty after drying of the disinfectant in order to avoid the accidental absorption of residues by the animals (FAD PReP, 2014). In case of ASF outbreak this period is 40 days (EC, 2002).
10.2.3 Disinfectants effective against ASFV
The choice of the disinfectant must take into consideration different aspects as the type of surfaces, the spectrum of activity, the efficacy and practicability under farm conditions (e.g. ease of handling, risk of corrosion of equipment, temperature stability), safety for operative staff, animals and the environment, costs, risk to store, etc. (Missouri Department of Agriculture, 2008; FAO, 2010). The preparation of disinfectant solutions must be performed by qualified operators strictly following the manufacturer's instructions (concentration, contact time, pH, temperature) (FAO, 2010). It is important to keep solutions clean and freshly made. Mixing disinfectants is inadvisable, as the potency of each may be nullified or a dangerous reaction may be caused, releasing heat or dangerous gases (CEREP, 2004).
There are no indications in literature regarding the ideal disinfectant against ASFV, but every country has approved and/or authorized a list of biocides effective against ASFV and thus only authorized biocides should be used and applied according to the producer’s instructions (Juszkiewicz et al., 2019). However, on the OIE website effective disinfectants against ASFV are reported (OIE, 2019). General knowledge of and experience in the use of disinfectants against enveloped viruses (CEREP, 2019; Gallina and Scagliarini, 2010; Krug et al., 2012; Krug et al., 2011; OIE, 2019; Shirai et al., 2000; Stone and Hess, 1973; Turner and Burton, 1997) have shown that the chemical compounds effective in inactivation of ASFV are:
- Formaldehyde 1%,
- Sodium hypochlorite (0.03% to 0.0075%),
- Caustic soda solution 2%,
- Glutaraldehyde, formic,
- Sodium or Calcium hydroxide 1% (effective at virus inactivation in slurry at 4°C),
- Phenols – lysol, lysephoform, and creolin,
- Chemical compounds based on lipid solvents,
- Multi-constituent compounds – Sodium chloride, Potassium peroxymonosulfate, ysoformin, Desoform, Octyldodeceth-20 (OD-20) surfactants, active substances, organic acids, glycosal, etc.
The use of some effective disinfectants against ASFV is limited due to their toxicity or safety (e.g. formaldehyde). In practice, only some of the chemical compounds mentioned above are contained in commercial disinfectants. The disinfectants recommended in the USA by The Environmental Protection Agency (EPA) are shown in Table 5. Currently there are commercial disinfectants based on phenolic and iodine compounds which are effective against the virus and can inactivate the ASFV at pH<4 and >11 (Gallardo et al., 2015; Geering et al., 2001).
Table 5. Disinfectants effective against ASFV (APHIS-USDA, 2011 modified; Geering et al., 2001)
Active ingredient(s) | Contact time | Application(s) |
Sodium chloride Potassium peroxymonosulfate | 10 min | In/on animal feeding equipment, livestock barns/pens/stalls/stables, livestock equipment, hog farrowing pen premises, hog barns/houses/pens, animal quarters, animal feeding and watering equipment, animal transportation vehicles, agricultural premises/equipment, human footwear |
Sodium dichloros-triazinetrione | 30 min | In/on animal living quarters, farm premises, shoe baths |
Sodium dichloros-triazinetrione | 30 min | In/on animal living quarters, farm premises, shoe bats |
Sodium dichloros-triazinetrione | 30 min | Animal quarters, animal feeding/watering, animal equipment, transportation vehicles |
Sodium dichloros-triazinetrione | 30 min | In/on livestock premises, animal feeding/watering equipment, animal equipment, animal transportation vehicles, farm premises, shoe baths. |
Sodium hypochlorite | 15 min nonporous 30 min porous | Indoor or outdoor use sites, such as agricultural, transportation, quarantine, and laboratory equipment and facilities; footwear/personal protective equipment. |
Citric acid | 15 min nonporous 30 min porous | Indoor or outdoor use sites such as agricultural and non-agricultural equipment and facilities; laboratory equipment and facilities; footwear/personal protective equipment, personnel decontamination. |
THE LIST OF DISINFECTANT PRODUCTS IS RECOMMENDED BY VEMEDIM TO PREVENT AFRICA SWINE FEVER
VIMEKON is a disinfectant with broad-spectrum activity, against gram-negative and gram-positive bacteria, fungi and virus.
Virus: IBDV, NDV, FMD virus, TGE virus, Hepatitisvirus, Avian influenza virus , PRRS virus.
Bacteria: Actinobacillus, Aeromonas, Bacillus, Bacteroides, Bordetella, Enterococcus, Erysipelothrix, E.coli, Haemophilus, Klebsiella, Listeria.
Fungi: Aspergillus, Candida, Fusarium , Trichophyton, Verticillium.
VIME-PROTEX is a Liquid disinfectant with A broad-spectrum activity against gram-negative and gram-positive bacteria, fungi and viruses.
- Virus: Newcastle Disease Virus (NDV), Infectious Bursal Disease Virus (IBDV) Avian Influenza Virus (AVI – H5N1), FMD virus, Infectious Bronchitis Virus, Pseudorabies Virus, Transmissible Gastroenteritis Virus, ...
- Bacteria: E.coli, Salmonella, Aspergillus, Pseudomonas spp, Klebsiella pneumoniae, Staphylococcus, Enterobacter aerogenes, Candida....
ALTAcid -The newest disinfectant generation. Broad-spectrum. High efficacy. Safety. Comfortable pine essential oil. Pest Repeller
A special combination between Quaternary Ammonium cation and Glutaraldehyde enhances the antibacterial spectrum. The antibacterial spectrum, which brings better disinfection efficacy, eliminate all susceptible viruses, bacteria, and fungi even in the presence of organic contaminants.
10.3 Vaccination
The lack of a vaccine limits options for ASF control because vaccination is widely accepted as the most effective way to control infectious diseases. Outstanding successes have included the global eradication of smallpox and rinderpest. However, failures include attempts to control ASF via vaccination in Spain and Portugal, because the live attenuated strains used caused unacceptable chronic disease post-immunization.
10.3.1. Desirable criteria for ASF virus vaccines
An EC report and recent reviews summarize progress toward a vaccine and necessary steps still to be completed. They concluded that good progress had been made but that a vaccine that could be used in the field would take several years. The desired characteristics for an ASF virus vaccine, elicited by expert opinion, include, most importantly, high efficacy in pigs of all ages and prevention of transmission of challenge virus, as well as safety in all age groups of pigs. A first-generation vaccine may not meet all of the other desired criteria but must meet the requirements of relevant regulatory authorities (Dixon et al., 2020).
10.3.2. Impact of vaccination on trade and diagnostic tests to distinguish infected from vaccinated animals
Reporting of ASF outbreaks, in disease-free regions, results in trade restrictions. Confirmation of freedom from disease is required to regain permission to export. Thus, a
critical factor in the decision to vaccinate is the disease status of the region and whether a diagnostic test is available to confirm freedom from disease. In regions where freedom from disease is not an issue, vaccination may also be used to reduce the burden of disease and prevent further spread. So-called differentiation of infected from vaccinated animal, or DIVA, tests can be used to monitor vaccine effectiveness, as well as to confirm freedom from disease. The best DIVA tests detect an antibody response to infection but not to the vaccine strain, such as those used in vaccines for Aujeszky’s disease. A DIVA diagnostic test may be used during the later stages of a vaccination campaign, when eradication becomes the goal. Thus, an efficacious ASF virus vaccine that prevents challenge virus replication and does not cause unacceptable clinical signs post-immunization is likely to be effective in many of the current epidemiological scenarios. Accompanying DIVA diagnostic tests may be developed and used later in the campaign (Dixon et al., 2020)
10.3.3. Vaccination of wild boar populations
Use of vaccines aimed at wild boar populations results in additional requirements. First, the vaccines must be immunogenic after oral administration and sufficiently stable in the external environment to maintain potency when exposed to extreme environmental factors. Second, for an oral immunization scheme to be feasible, a suitable delivery device in the form of bait is needed. These baits must be stable; effective in reaching individuals of different sexes and ages; and traceable and safe for wild boar, as well as for non-target species and the environment. Meeting all these requirements in a marketable product will be challenging in terms of experimental studies and field trials, as well as costly and time consuming. However, vaccination has been used successfully to control classical swine fever disease in wild boar via delivery of live attenuated vaccine in baits. For this reason, the prospect for oral ASF virus vaccination of wild boar using baits is considered good (Dixon et al., 2020).
10.3.4. Vaccines for ASF virus: state of play
The complexity of the ASF virus genome and virus particles has been a major factor in delaying vaccine development. Inactivated virus particles fail to protect against ASF virus challenge. Observations that pigs that recover from infection with less virulent isolates were protected against challenge with related virulent virus showed that vaccination was possible and that live attenuated vaccines were most likely to be successful within a shorter time frame. Correlates for protection are poorly characterized, and depletion of CD8+ cells showed that these are required for live attenuated virus induced protection. Antibodies also have a role in protection. The key virus antigens involved in protection have not been fully characterized (Dixon et al., 2020).
10.3.5. Live attenuated vaccines
Several live attenuated ASF virus vaccine candidates, either produced by passage in cell culture or naturally occurring, induce good protection but cause unacceptable adverse clinical reactions, including a chronic form of disease in some vaccinated pigs. Increasing knowledge of the functions of ASF virus-encoded genes has opened a route for
targeted gene deletions to produce rationally attenuated ASF virus vaccines. Deletion of genes for inhibitors of the type I interferon response results in attenuation of virulent virus and induction of protection against challenge, with little or no apparent replication of challenge virus. Importantly for the eventual commercial development of vaccines, deletion of these genes does not reduce virus replication in cells, so high titers can be obtained in culture (Dixon et al., 2020).
10.3.6. Subunit vaccines
The development of subunit vaccines, defined as those that deliver a proportion of the virus, has lagged behind that of live attenuated vaccines owing to the need to identify potentially protective antigens. Recombinant proteins of p30/CP204L, p54/E183L, and CD2v/EP402R have been shown to confer partial protection in some studies. DNA vaccination with a library expressing multiple small-virus DNA fragments or pools of host-restricted or defective virus vectors (for example, Modified Vaccinia Ankara or human adenovirus) expressing ASF virus antigens induced partial protection (Dixon et al., 2020).
11. References
Alejo, A., Matamoros, T., Guerra, M., Andrés, G., 2018. A proteomic atlas of the African swine fever virus particle. Journal of virology 92, e01293-01218.
Alonso, C., Borca, M., Dixon, L., Revilla, Y., Rodriguez, F., Escribano, J.M., 2018. ICTV virus taxonomy profile: Asfarviridae. Journal of General Virology 99, 613-614.
Beltran-Alcrudo, D., Gallardo, M., Kramer, S., Penrith, M., Kamata, A., Wiersma, L., 2017. African swine fever: detection and diagnosis. Food and Agriculture Organization of the United Nations (FAO).
Chenais, E., Depner, K., Guberti, V., Dietze, K., Viltrop, A., Ståhl, K., 2019. Epidemiological considerations on African swine fever in Europe 2014–2018. Porcine health management 5, 1-10.
De Lorenzi, G., Borella, L., Alborali, G. L., Prodanov-Radulović, J., Štukelj, M., & Bellini, S. (2020). African swine fever: A review of cleaning and disinfection procedures in commercial pig holdings. Research in Veterinary Science, 132, 262-267.
Dixon, L., Islam, M., Nash, R., Reis, A., 2019a. African swine fever virus evasion of host defences. Virus research 266, 25-33.
Dixon, L., Sun, H., Roberts, H., 2019b. African swine fever. Antiviral research 165, 34-41.
Dixon, L.K., Stahl, K., Jori, F., Vial, L., Pfeiffer, D.U., 2020. African swine fever epidemiology and control. Annual Review of Animal Biosciences 8, 221-246.
Galindo, I., Alonso, C., 2017. African swine fever virus: a review. Viruses 9, 103.
Gaudreault, N.N., Madden, D.W., Wilson, W.C., Trujillo, J.D., Richt, J.A., 2020. African swine fever virus: an emerging DNA arbovirus. Frontiers in Veterinary Science 7, 215.
Maclachlan, N.J., Dubovi, E.J., 2010. Fenner's veterinary virology. Academic press.
Netherton, C.L., Connell, S., Benfield, C.T., Dixon, L.K., 2019. The genetics of life and death: virus-host interactions underpinning resistance to African swine fever, a viral hemorrhagic disease. Frontiers in genetics 10, 402.
Salguero, F.J., 2020. Comparative pathology and pathogenesis of African swine fever infection in swine. Frontiers in Veterinary Science 7, 282.
Sánchez-Vizcaíno, J., Mur, L., Gomez-Villamandos, J., Carrasco, L., 2015. An update on the epidemiology and pathology of African swine fever. Journal of comparative pathology 152, 9-21.
Sánchez‐Vizcaíno, J.M., Laddomada, A., Arias, M.L., 2019. African swine fever virus. Diseases of swine, 443-452.
Schulz, K., Staubach, C., Blome, S., 2017. African and classical swine fever: similarities, differences and epidemiological consequences. Veterinary research 48, 1-13.
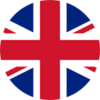